Game Changers from Nuclear Technology
We begin by examining the possible advances in nuclear science and technology that could alter the future of nuclear power. The nuclear fuel cycle—mining, enrichment, reactor operation, and reprocessing, storage, or disposal—provides a convenient organizing framework for this discussion. A full discussion of the intricacies of the fuel cycle is beyond the scope of this paper, and interested readers can consult one of the many excellent existing reviews.22
Mining and Milling
The first steps in the nuclear fuel cycle are the mining and milling of natural uranium. The most obvious potential game changer at this stage is a shortage of recoverable natural uranium, but we do not deem this a likely eventuality. Should current resources prove uneconomical, new extraction technologies or even fuel cycles may be implemented. However, since fuel extraction and fabrication costs represent a negligible contribution to the overall economics of nuclear power, it is difficult to see a game changer at this stage.
Is the current worldwide nuclear energy capacity sustainable? Are current resources sufficient to fuel increased global demand for nuclear power? To operate a 1 GW reactor for one year, about 170 tons of natural uranium are needed, assuming a once-through cycle and thermal efficiency of around one-third. The total world usage is around 65 kilotons per year. The amount of uranium mined annually accounts for only two-thirds of this world nuclear demand, with the shortfall made up by reenriched depleted tails, repurposed military uranium, and reprocessed nuclear fuel. Figure 2 shows the geographical distribution of known recoverable resources according to the report Uranium 2009 (the Red Book).23
Figure 2: Known Recoverable Resources of Uranium, in Tons, as of 2009
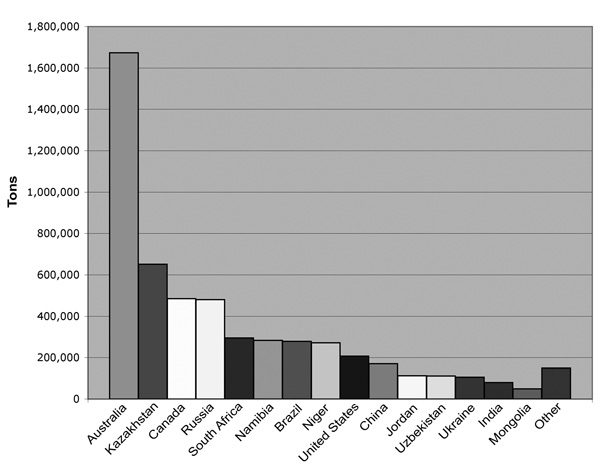
Source: Figure created by authors based on data from Uranium 2009: Resources, Production, and Demand (Nuclear Energy Agency/Organisation for Economic Co-operation and Development, 2011).
The figure does not take into account the ore grade, which ranges from 20 percent in certain deposits in Canada to less than two-millionths of a percent in sedimentary rock. Energy costs to extract reasonably pure uranium increase with decreasing grade. However, the use of even very low-grade ore does not significantly increase the cost of nuclear power per kilowatt-hour, which is relatively insensitive to the price of natural uranium. In fact, fuel costs for nuclear power are proportionally lower than for other major energy sources, as shown in Table 2.
Table 2: Total Levelized Cost and Variable Operation and Maintenance (O&M) Cost (Principally Fuel Cost) for Electricity Generating Technologies Brought Online in 2016, by Cost per Megawatt-Hour (MWh)
Plant Type | Total Levelized Cost ($/MWh) | Variable O&M including Fuel |
Coal (conventional to advanced with carbon capture and sequestration) |
100–130 | 24–26 |
Gas (Combined Cycle) | 80 | 55 |
Advanced Nuclear | 119 | 9 |
Wind | 150–190 | 0 |
Solar Photovoltaic | 400 | 0 |
Solar Thermal | 250 | 0 |
Geothermal | 116 | 0 |
Biomass | 111 | 25 |
Hydro | 120 | 7 |
The raw material for nuclear power is not a renewable resource, and concerns have been raised24 that future exploration may yield deposits that are uneconomical to extract or are sufficiently low-grade to require an unacceptable investment of energy to separate pure uranium. However, we view this eventuality as rather unlikely: if uranium is scarce, there will be more incentive to look for it. Deposits that have thus far been uneconomical to extract may become attractive in a high-demand future. In addition, the arguments in Jan van Leeuwen and Philip Smith rely on the assumption that all high-grade deposits have already been identified and exploited and that all subsequent deposits will be extremely low-grade; recent discoveries of high-grade ore in Canada cast doubt on the accuracy of this assumption. A 2003 MIT study25 found that known recoverable resources of uranium appear to be sufficient to support a modest increase in nuclear construction over the next century; we agree with this analysis and do not see a natural resource shortage as a likely game changer.
Projections of uranium availability consider global resources, yet in the real nuclear market, national boundaries matter, as do such international commitments as the obligations of state-parties to the NPT and those undertaken by members of the Nuclear Suppliers Group (NSG). For reasons of energy security, countries may not wish to rely on imported uranium. India in particular was denied access to the international uranium market until very recently and has very little indigenous natural uranium. It does, however, have large deposits of thorium, which can be used to breed fissile U-233 isotopes. Because thorium is fairly abundant worldwide, if India succeeds in both developing and exporting this technology, it could effectively remove both its own resource constraints and uranium availability in general from the nuclear energy equation. Now that India has access to the international uranium ore market it is uncertain to what extent it will make the investments needed to use thorium technologies.
There is also the possibility of developing alternatives in extracting uranium from seawater. Seawater contains about three parts per billion of uranium. While this is a tiny concentration, the sheer volume of the oceans means that up to 4 billion tons of uranium may be extracted from seawater, enough to sustain ten times current consumption for more than six thousand years. While this method is not used commercially at present, a Japanese team has succeeded at extracting uranium from seawater at about three times the cost of mining. This method could prove attractive to countries without indigenous uranium resources, and if it can be done with minimal environmental impact, it could be a viable alternative to mining. Still, economical extraction from seawater is likely to be a game changer only in the limited area of uranium resource exploitation. It is unlikely to change the game for nuclear power as a whole.
Enrichment
Because enrichment is a complex process and poses an inherent proliferation danger, game changers in the enrichment stage of the nuclear fuel cycle are possible in many dimensions. On the technical side, new enrichment processes may be game changers not because they are easy to conceal, as conventional wisdom holds, but because they may be implementable on small scales. On the economic side, the entry of new suppliers into the enrichment market could change the game, as could a concerted geopolitical effort to manage the proliferation risk posed by the technology.
In order to discuss game changers for enrichment, it helps to understand the current state of both technology and the market. Very few reactors run on natural uranium; after mining and milling, most nuclear power plants must then undertake enrichment to increase the concentration of the fissile isotope U-235 relative to the naturally abundant isotope U-238. In the previous section, we showed that the economics of nuclear power are relatively insensitive to the costs of raw uranium; likewise, the enrichment process adds relatively little to the cost per kilowatt-hour. At present, enrichment services are provided to the international market by a few dominant players. France, the United States, and Russia, along with URENCO, are the major international providers of enrichment services. In addition, a number of other countries have (or have had) enrichment facilities for domestic purposes; more have either indicated their interest in acquiring this capability or are in the process of acquiring it. Current world enrichment capacity exceeds current world demand, making for a competitive market, albeit one heavily constrained by suppliers’ agreements. While the vast majority of power reactors use low enriched (near or below 5 percent) uranium, which cannot be used directly for nuclear weapons, once a country acquires the capability to enrich to this level it is comparatively simple to achieve the high enrichment required for weapons-grade uranium. Enough highly enriched (at or exceeding 90 percent) uranium for a nuclear weapons requires only a small percentage of the separative work needed to provide fuel for a standard power reactor for a year. Thus, enrichment facilities are considered sensitive from the standpoint of nuclear proliferation.
Currently, a large proportion (two-thirds and growing) of enrichment is accomplished by cascades of centrifuges, which separate isotopes by means of the centrifugal force. Global Laser Enrichment, a joint venture of General Electric, Hitachi, and Cameco, has recently met with some initial success in an enrichment plant that would rely on the separation of isotopes by selective laser excitation of the electrons of isotopes, a process known as SILEX. If a laser enrichment plant is successfully developed to full scale, this method could provide cheaper and, in some ways, less technically demanding enrichment services than the currently dominant centrifuge-based enrichment plants. Some argue that this process, unlike large centrifuge projects, can be more easily concealed and therefore poses a new proliferation threat. However, clandestine enrichment is not necessarily a game changer per se. Technologies that are easy to hide already exist: Iraq’s clandestine enrichment program, for example, involved a calutron mass spectrometer, one of the oldest enrichment technologies in existence. In order to constitute a true game changer from the standpoint of proliferation, a new enrichment technology must be not only concealable but also smaller in scale and simpler to implement. Current enrichment technologies are available only on large scales; therefore, proliferation requires active involvement at the state level. It is not clear whether SILEX would lead to changes in this respect. An enrichment process that makes enrichment available to sub-state actors, potentially posing unacceptable terrorism risks, could have a profound impact on how nuclear power is regulated and exported in the future.
Even without a new technology, if some current trends continue, the nuclear fuel enrichment market could look very different in a few years than it does now. At least two such trends are evident. One is the attempt by countries rich in uranium ore, such as Australia, Mongolia, and Kazakhstan, to add value to their uranium exports by building the conversion and enrichment facilities needed to export enriched nuclear fuel. The other is the reactivating or upgrading of enrichment facilities outside the major exporting countries. For instance, Argentina, Brazil, India, Iran, Pakistan, South Africa, and South Korea all are either reactivating facilities, upgrading ongoing facilities, experimenting with separation technologies, or negotiating the necessary agreements to enable them to start an enrichment process. None of these countries, except perhaps South Africa and eventually South Korea, is likely to be able to compete on price with existing large-scale suppliers of enrichment services in the short or medium term, but the efforts may continue for strategic and developmental reasons. Moreover, the loss of the present oligopoly could make the enforcement of nuclear exports guidelines difficult or irrelevant or, alternatively, lead to more multinational, perhaps regional, facilities. The latter course is more desirable from the standpoint of inhibiting proliferation and terrorism, but it requires the states involved to accept some internationally agreed constraints. Such acceptance will hinge on a variety of local circumstances, but in our view, one factor is likely to be generally important: the international agreement must retain the competitiveness that characterizes the present enrichment market, where the United States, URENCO, France, and Russia are competing, with China and others as possible suppliers in the future.
A number of proposals have been put forward to lessen the risk associated with the spread of enrichment technology.26 The proposals range from internationalization or further multinationalization of the facilities to a freeze on the number of states that have such facilities. Little agreement has been reached. States have been reluctant to give up their ability either to buy enrichment services in a competitive market or to overcome any present or future opposition to their fueling nuclear reactors. Acceptance will therefore depend on both economic and political dimensions of any proposal. On the economic side, clients of multinational or regional facilities must be satisfied that they could not buy enrichment services more inexpensively or otherwise on better economic terms elsewhere. By the same token, the international agreement must not constitute a barrier to entry for other, potentially more competitive suppliers or for new technologies. On the political side, clients must be satisfied that their political relations with the sponsors of some facilities will not interfere with their ability to purchase enrichment services from other facilities, assuming that the constraints related to preventing proliferation or terrorism are met in all cases. In other words, disputes on grounds having nothing to do with the utilization of nuclear energy, such as those concerning commercial arrangements, territory, or human rights, must not result in curtailed access to enrichment facilities.
Some of the solutions presented to date (freezing the number of states that can provide enrichment services or relying on a single international authority) do not meet these minimum criteria, but there is no a priori reason why other solutions could not meet them.
Reactors
For nuclear reactors, meaningful game changers stemming from technological developments would address three main problems: high initial costs, generation of spent fuel that contains plutonium, and high radiotoxicity of waste products.
Again, it is useful to review the current state of reactor technology and economics. Most nuclear power reactors in the world today are variations on a single basic design, the light water cooled and moderated reactor (LWR). LWRs use low enriched uranium (LEU, typically 4 to 5 percent U-235). With LEU, it is impossible to sustain the chain reaction that leads to a nuclear explosion: weapons require highly enriched uranium (HEU), with concentrations up to and exceeding 90 percent U-235.
These current power reactors have a thermal efficiency around 33 percent and a capacity factor ranging up to and a little more than 90 percent when operated in base-load mode. They have excellent safety records, in part because the greatest risk to workers and the public comes from mining and transportation and also because even the worst accident for this type of reactor (the Three Mile Island accident in the United States in 1979) did not lead to any significant off-site damage to people and property. Nuclear power can be competitive with other means of generating electricity, depending on the cost of capital, the length of time for licensing and construction, and factors having to do with competing technologies. Nuclear reactors emit less than a tenth of the greenhouse gases emitted by coal-fired generators of the same size, including emissions during construction, mining, transportation, operation, and shutdown; they emit little or no other pollutants. The radioactivity emitted throughout the nuclear fuel cycle in particular, including operations, is less than what is released from coal mining operations and coal combustion.
Further improvements in efficiency and safety, as well as work toward additional lifetime extensions, are either ongoing or planned; these incremental developments lie well within planning horizons and cannot be considered game changers. Greater efficiency requires materials that can withstand more radiation and higher temperature, allowing the nuclear fuel to stay in a reactor longer and burn a higher fraction of its fuel and leading to fewer interruptions for refueling. Higher temperatures would increase the ratio of electric power to heat loss. The key to improved safety (besides better operator training and regulatory compliance, which are not technical but are the main contributors to safety) lies with more “passive” safety devices, ones that activate when needed without human or electrical intervention.
In the past, the expansion of nuclear power has been accompanied by real and imagined proliferation threats. Plutonium in spent fuel, while not ideal for weapons, can in theory be separated and used to build a bomb. Some of the ideas currently considered by reactor builders and governments would reduce but not eliminate these fuel diversion concerns. Others would change the nature of the current fuel cycle, either by relying more on plutonium separation for their fuel or by using thorium, which involves a different fuel-cycle. These technological changes give rise to political and security challenges, which we consider in the next section. The impact of very advanced concepts that have been studied only on paper or with computer modeling, such as the traveling wave reactor, is difficult to envisage; however, it is unlikely that even spectacular new designs will lead to nuclear dominance in the electricity sector globally unless these designs changed what are seen as the downside factors for nuclear power: namely, the high front-end investment cost, the generation and disposal of radioactive materials, and popular perceptions of its safety and security.
Small modular reactors (SMRs) could alleviate the first of these problems but only at a cost and for much smaller applications than nuclear power plants’ current designs allow. Models close to commercial availability range from 45 to 125 MWe, and they cost far less than standard-size reactors, are more adaptable to less capable grids and lower demand sites, do not require as many scarce specialized vendors, and require fewer refuelings during their lifetimes. On the other hand, a number of regulatory issues remain to be settled, SMRs cost more on a dollar-per-megawatt basis than larger reactors, and the first models would pose the usual “first of a kind” issues. These reactors may also displace smaller-capacity renewables or pose increased security and transportation problems, as spent fuel must be stored at or transported to more locations. Perhaps the biggest drawback of SMRs is the increased potential for accidents: while technology is modular and exportable, safety culture seldom is. These challenges notwithstanding, three utilities have so far committed to getting one such unit approved for commercial use in the United States.
On a much longer timescale, several advanced designs that would alleviate at least one of the problems listed above are under investigation. These designs include new versions of ideas abandoned when LWRs were first commercialized, such as sodium-cooled or lead-bismuth cooled reactors with extended fuel cycles, gas-cooled reactors, pebble-bed fuel reactors, and thorium-based reactors, as well as new ideas, including the traveling wave reactor (TWR). This design, if realized, promises to extend fuel life to forty to sixty years with no enrichment (other than an initial fuel load) or reprocessing, and to run on depleted uranium. A commercial version of the TWR would likely require further research into new materials capable of withstanding high temperatures and neutron fluence.
Inexpensive, viable, and sustained nuclear fusion, should it become reality, would drastically change the game for nuclear power. There are two main approaches to sustained fusion: magnetic confinement, in which the fusion plasma is held in place by a magnetic field, and inertial confinement, in which a fuel target is heated and compressed until light elements can fuse. Both approaches are the subject of large-scale scientific investigations. The International Thermonuclear Experimental Reactor (ITER) facility in Cadarache, France, utilizes magnetic confinement and is scheduled to come online in the 2020s. The Lawrence Livermore National Laboratory in California investigates laser fusion at its National Ignition Facility (NIF), and there are other smaller efforts elsewhere in that direction. Some of the underlying physics has now been tested, notably in the case of magnetic confinement, and the NIF may be a year or two away from igniting a small amount of nuclear fuel. But in both cases, important scientific and materials questions remain outstanding. Commercial fusion power of either type, even in a best-case scenario, is probably decades away. If successful, however, this technology would do away with waste and proliferation issues as we know them. Viable, economical fusion would likely be a game changer, not just for nuclear fission but for the entire energy sector.
The Back End of the Fuel Cycle
The back end of the fuel cycle is perhaps the most politically contentious problem in nuclear energy. Spent fuel is comprised of about 95 percent unburned natural uranium, which is not particularly radioactive and could be handled safely. However, a further 4 percent is composed of fission fragments, most of which remain dangerously radioactive for nearly five hundred years. The remaining fraction consists of very heavy elements, including plutonium, that have been created along with the fission process and the U-235 that has not been fissioned. These elements, along with certain fission products, constitute high-level waste and will remain highly radioactive for hundreds of thousands of years. Figure 3 shows how the radiotoxicity of spent fuel elements changes over time. (To provide some context: a few sievert over the human body will cause severe radiation disease or death.) In 2009, U.S. nuclear plants generated about 0.7 terawatt-years.
Figure 3: Radiotoxicity Inventory, in Sievert per Terawatt-Hour (Sv/TWhe), in Spent Nuclear Fuel at Ten Years and Beyond
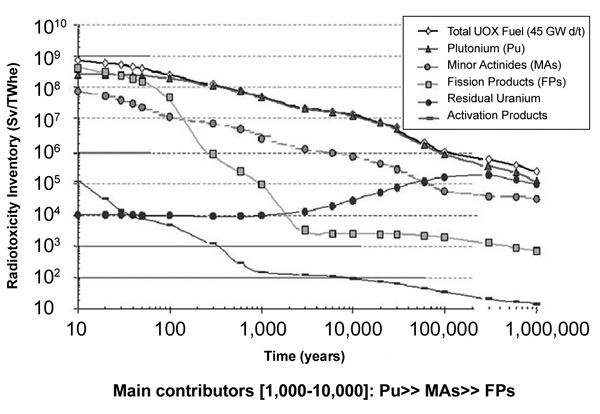
Source: Charles Madic et al., “Futuristic Back-End of the Nuclear Fuel Cycle with the Partitioning of Minor Actinides,” Journal of Alloys and Compounds 444–445 (2007): 23–27. Figure reprinted here with permission from Elsevier Ltd.
At present, there are two methods for handling this spent fuel. One, strongly advocated and expensively pursued by the United States, is the so-called once-through cycle: after the first pass through the reactor, spent fuel is not reused for its remaining energy content but, after a cooling period of years, is sent to an underground depository and buried irreversibly. Several other countries, notably Finland and Sweden, are also pursuing this method. The U.S. policy is now in limbo as a result of the Obama administration’s 2009 decision to abandon the chosen repository site of Yucca Mountain in Nevada, after some twenty years and $20 billion of investigation and investment. This was arguably a purely political decision, and it is not inconceivable that it may be reversed. In the meantime, spent fuel is stored in sealed casks at utility sites after an initial cooling period of several years.
A variation on this approach has been adopted by Russia, which is accepting spent fuel and other high-level waste from other countries for long-term storage and possible disposal. Russia, along with France, anticipates that spent fuel may acquire commercial value in the future.
The other approach, once pioneered in the United States but now used in France, Russia, Japan, and some other countries,27 is to reuse or recycle the spent fuel in order to utilize its plutonium content. Most American observers, unlike their French and Russian counterparts, consider this method to be more expensive than the once-through method, given present prices of uranium. The comparison is complicated for a number of reasons, including different sunk costs and different economic assumptions. Furthermore, the comparison is not perfect because separating the plutonium and other actinides makes the remaining waste smaller in volume and less radioactive after some time.
Two factors increase the urgency of the spent fuel debate. First, because of local political opposition, most countries find it difficult to site spent fuel depositories. As a result, nuclear exporter countries or firms that could offer “cradle to grave” programs, whereby the importing country would buy, in one package, the reactor, fueling services for its lifetime, and disposal of spent fuel outside the country, are likely to have an advantage in selling reactors and fuel services. This issue is discussed further in a subsequent section on the changing nuclear market. The other factor stems from the possible utility of the plutonium in spent fuel for weapons. As noted in an earlier footnote, this “reactor-grade plutonium” is usually not well suited to weapons use because of its high radioactivity, but weaponization is theoretically possible. Expansion of nuclear power may therefore constitute a diversion danger for countries or groups that have access to a plutonium separation plant.
Technological game changers at the back end of the nuclear fuel cycle could therefore include new recycling methods that do not separate the plutonium in spent fuel from its radioactive matrix, reducing the chance it could be diverted to weapons use. While such innovations in reprocessing have been considered in the past, none have been commercialized, and it is likely that they would require new fuel and reactor designs as well. The most likely game changers at the back end include new models of viable storage and disposal or technology that helps facilitate cradle-to-grave packages. These developments, while they may be aided by new technology, are perhaps more a function of the political and market forces we discuss later.
Accidents
The potential of accidents to alter the no-surprise scenario depends on many factors: the developmental stage at which they occur, their consequences, and the public perception of nuclear power at the time they occur. Early accidents, mainly in developmental facilities, did not affect the growth of nuclear power, which at the time was popularly supported. The Three Mile Island accident, which caused no casualties, and Chernobyl, which did, had large political impact. However, these accidents came at a time when the growth of nuclear power had ceased in both the United States and Russia. Therefore, they caused little change in actual planned investments, although they had a devastating impact on the anticipated future role of nuclear power in the United States and Europe.
Things can go wrong at any stage of the nuclear fuel cycle. Mines, enrichment plants, reactors, separation plants, and storage and disposal sites are all vulnerable to accidents of varying degrees of severity. The term accident is used in the industry for a wide variety of events, ranging from some that affect only a single facility to some that may result in serious damage to the population, environment, or economy. The accidents most likely to change the nuclear game are those that are both severe and peculiarly “nuclear”: that is, those arising not in the mining or transportation categories, but rather at reactors, at the storage sites used in the early years after spent nuclear fuel is removed from the reactors, or at recycling plants.
Nuclear power plants are complex technological systems of interacting parts that also require interactions with human operators. Such complex systems are subject to what Perrow (cited above) calls “normal accidents”: the culmination of interlinked technical failures compounded by human error. These incidents, while impossible to predict and difficult to prevent, are not low-probability outliers: they are dangers inherent in complex technology. Accidents must therefore be expected over the fifty-year time horizon of this study. They have certainly occurred over the past sixty years, although the accident rate and consequent damage to health and environment have been lower for nuclear energy than for hydrocarbon generation. The accidents were almost always due to human error compounded by the complexity of the technology.
Accidents can have societal, financial, and environmental and health consequences. Significant environmental and health consequences have been extremely rare after the early days with the important exception of Chernobyl, which involved a reactor design that would not have met Western safety criteria. Societal consequences have been more frequent, as exemplified by the 1979 Three Mile Island accident, which focused attention on reactor operator training and compounded a growing sense of disenchantment with nuclear power in the United States. Lesser accidents elsewhere in the United States and the world have also had a negative impact on the general perception of nuclear power, which is still seen as a dangerous unknown by the media and much of the public. The financial consequences of both actual accidents and serious infractions of governmental safety rules have been severe. The Three Mile Island accident is estimated to have cost its operator well over $2 billion, and several other accidents have cost their operators over $1 billion.28 Even a near-accident, such as erosion in a core component discovered, in 2002, at First Energy’s Davis-Besse plant in Ohio, can cost an operator over $600 million.29 The financial costs of accidents and infractions make potential investors wary, but their impact on plans for the future depends heavily on the economic and political environments in which they occur.
While technological advances and improvements in regulation and training have made a major difference, it is prudent and realistic to expect accidents and to develop contingency plans to minimize their consequences. A principal concern in this regard is the possible expansion of nuclear power to new users and new exporters. The most significant way to minimize accidents and to alleviate their consequences if they occur is to adopt a safety culture at every step required in the construction and operation of nuclear facilities. Safety can be a hard sell to new users: it is an added cost and often a factor in slowing operations. Delivery of a certified design unit to an approved site is only the first step: regulators must maintain a presence in-country, personnel must be trained to report problems to regulators (and be protected when they do so), and management must be trained to deal with problems openly. The International Atomic Energy Agency (IAEA) can play a crucial role, especially at the time of expansion to new users, yet it has not received the additional budget and political support it needs.30
Former chairman of the U.S. Nuclear Regulatory Commission, Richard Meserve, notes that the present international safety regime is an ad hoc mixture of intergovernmental organizations (of which the IAEA is the most prominent), several multinational networks, and stakeholders in the international nuclear industry held together by “a framework of international conventions, international safety standards, codes of conduct, joint projects, and international conferences and workshops.”31 Licensing and operational standards for individual projects remain under national authority. Meserve rejects the idea of an international regulator that would displace national authorities, but he proposes a number of steps to improve the existing regime, including strengthening international safety and security services, broadening communication networks for sharing operational practices and incident information, and improving harmonization and international evaluation of designs and practices. Implementing such recommendations becomes increasingly important as the number of new entrants to nuclear power grows.
The nuclear industry throughout the world is vulnerable to accidents, including those impacting health and the environment. Changes such as those advocated by Meserve, together with a well-cultivated safety culture, will help minimize both the frequency and severity of accidents and mitigate their consequences when they occur. An accident of even moderate severity, coupled with a political culture of ambivalence or nervousness regarding nuclear power, represents a serious potential game changer.
ENDNOTES
25. Craig, Gadgil, and Koomey, “What Can History Teach Us?”
26. Such proposals include those made by the Bush administration, “President Announces New Measures to Counter the Threat of WMD,” a fact sheet issued by the White House, February 11, 2004, http://www.whitehouse.gov/news/releases/2004/02/20040211-4.html; by Mohammed ElBaradei when he was Director General of the International Atomic Energy Agency, published as “Towards a Safer World,” The Economist, October 16, 2003, http://www.nuclearfiles.org/menu/key-issues/nuclear-weapons/issues/proliferation/fuel-cycle/elbaradei-economist.htm; and by several authors, including Chaim Braun and Michael May, “An International Regime of Fresh Fuel Supply and Spent Fuel Disposal,” The Nonproliferation Review 13 (1) (March 2006). In particular, see the recent review by Pierre Goldschmidt, “Multilateral Nuclear Fuel Supply Guarantees & Spent Fuel Management: What are the Priorities?” Daedalus 139 (1) (Winter 2010): 7–19.
27. See http://www.world-nuclear.org/info/inf69.html for a list of countries as of 2008. China is claiming it also can and will reprocess nuclear fuel.
29. See http://www.ohio.com/business/87712397.html.
30. For an authoritative review of this issue, see the report from the Commission of Eminent Persons, chaired by Ernesto Zedillo and commissioned by the IAEA, Reinforcing the Global Nuclear Order for Peace and Prosperity: The Role of the IAEA to 2020 and Beyond. The report recommends that IAEA members should allocate the organization “considerably larger resources”; see http://www.iaea.org/newscenter/news/2008/2020report.html.