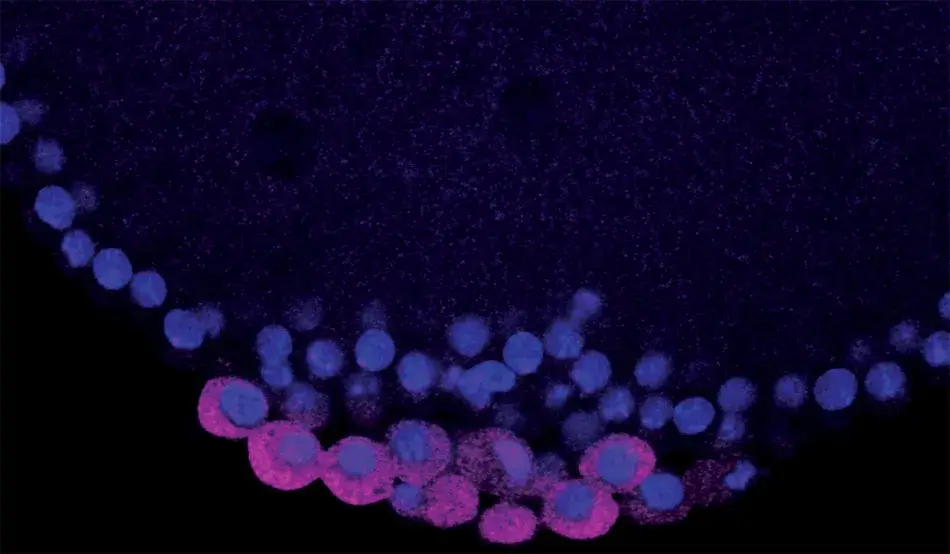
The Academy’s Francis Amory Prize recognizes major contributions to the field of reproductive biology and was first awarded in 1940. Over the years, the prize recipients have reflected the increasing complexity and remarkable scientific progress in the field of reproductive biology.
The Academy awarded the 2020 Francis Amory Prize in Reproductive Medicine and Reproductive Physiology to Ruth Lehmann and Gertrud M. Schüpbach in recognition of their extraordinary research that has advanced the areas of DNA repair, embryonic development, RNA regulation, and stem cell research.
The virtual program featured presentations by Gertrud Schüpbach and Ruth Lehmann, and included remarks by Academy President David Oxtoby, an introduction by Princeton University President Emerita Shirley Tilghman, and a reading of the Francis Amory Prize citations by Chair of the Academy’s Board Nancy C. Andrews. The introduction and an edited version of the presentations follow.
2096th Stated Meeting | February 3, 2021 | Virtual Event
Shirley Tilghman is President Emerita and Professor of Molecular Biology and Public Affairs at Princeton University. She was elected a Fellow of the American Academy of Arts and Sciences in 1990 and serves as a member of the Academy’s Board of Directors.
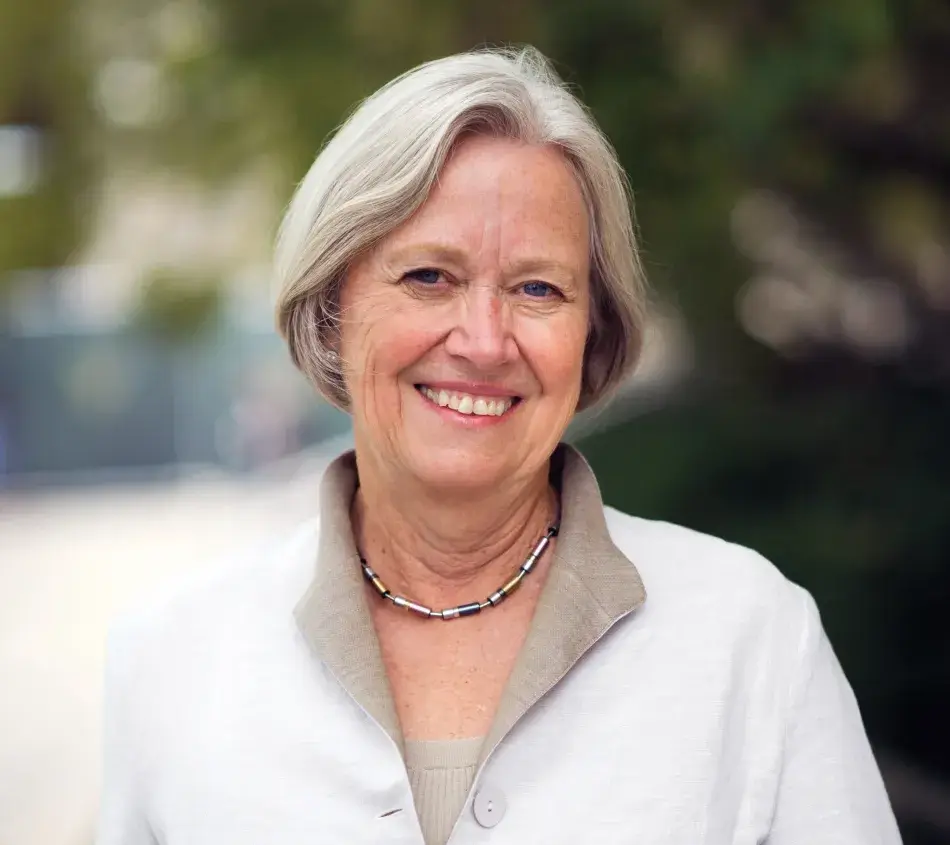
Introduction
It is my great pleasure to introduce this year’s recipients of the Francis Amory Prize: Gertrud Schüpbach, who is known to all as Trudi, and Ruth Lehmann. They have each made seminal contributions to our understanding of one of the most fundamental questions in developmental biology: how does an early embryo assign identities to its cells so that they learn their future fates? For their organism of choice, Drosophila melanogaster, the earliest of those decisions are determined by the mother, prior to fertilization, while the egg is developing in the mother’s ovary. The egg is prepackaged with information that specifies anterior versus posterior, dorsal versus ventral – setting up in other words which end is up and which is down, which side is back and which is front. In organisms like Drosophila, in which survival requires development to proceed as rapidly as possible, a strategy that prepackages as much information as possible in the egg makes perfect evolutionary sense.
As a young scientist at Princeton University, Trudi made one of her most important and lasting contributions to science. With her husband and collaborator Eric Wieschaus, she conducted a large genetic screen in Drosophila to identify the genes that act in the ovary of the mother to pattern the developing oocyte. These genes, called maternal effect genes because they act in the mother and not the embryo, were given colorful names such as torpedo, gurken, torso, trunk, vasa, staufen, and tudor – names that described the aberrant appearance of embryos born from mutant mothers.
By pointing to the importance of these genes, Trudi and Eric created a bonanza for the developmental biology community, whose members eagerly jumped on individual genes to study them in detail and uncover their specific roles in patterning the embryo. Trudi’s genetic screens are beautiful examples of a scientific achievement that catalyzed a whole new direction for a field.
Trudi chose to study the genes that act earliest in the patterning of the oocyte by focusing on those that affect the appearance of the eggshell as well as the resulting embryo. The developing egg begins as a single cell, which divides four times to produce sixteen cells, one of which will be designated as the future oocyte, while the rest become nurse cells that cater to the oocyte as it develops. These cells are surrounded by follicle cells that were not thought to be particularly relevant in the development of the egg until Trudi’s lab showed that they in fact play an essential role in both anterior-posterior and dorsal-ventral polarity through cell-to-cell communication with the developing oocyte.
Using elegant genetic and molecular approaches, Trudi’s lab showed that the follicle cells communicate through a protein encoded by a maternal effect gene called torpedo that receives a signal produced in the oocyte, the product of the maternal effect gurken gene. When either is mutated in the mother, her embryos are unable to develop dorsal structures. When torpedo and gurken were cloned, it was revealed that they belonged to an important family of signaling proteins that we now know play many roles throughout eukaryotic biology, and they have been implicated in cancer when they are mutated or mis-expressed.
But the story does not end there. How does the signaling produce dorsal-ventral asymmetry? During early oogenesis, Trudi demonstrated that the messenger RNA for gurken becomes localized to the future dorsal side of the oocyte, tightly associated with the oocyte nucleus. She then showed that the localized gurken RNA produces a high concentration of Gurken protein exclusively on one side of the egg, sending the signal that this will be the future dorsal side of the embryo. But timing is everything in biology, so localization alone is not sufficient to send the Gurken signal at precisely the right time. Trudi has now shown that the gurken RNA is not just localized, but its translation into Gurken protein is tightly regulated as well. Thus, with a remarkable combination of genetic and biochemical insights, Trudi brought the story of the initiation of dorsal patterning full circle.
After a dazzling career, Trudi has recently retired as the Henry Fairfield Osborn Professor of Molecular Biology at Princeton University. At Princeton, she is a highly regarded and beloved teacher of genetics to both undergraduate and graduate students, and she has mentored many students and fellows in her lab who have gone on to have successful careers in science. A former Howard Hughes Medical Institute Investigator and member of both the National Academy of Sciences and the American Academy of Arts and Sciences, Trudi received the Edwin F. Conklin Medal from the Society of Developmental Biology in 2006 and an honorary Ph.D. from the University of Zurich in 2011. She served the scientific community in many ways, including as the president of the Genetics Society of America in 2008 and as the president of the Drosophila Board in 2000. I cannot think of anyone more deserving of this award.
The second Amory Award recipient is Ruth Lehmann. Ruth’s interests lie both upstream and downstream of Trudi’s, as she is interested in how germ cells, those cells set aside in early embryogenesis that are destined to become the future sperm or eggs, are specified and how they migrate to the gonad and assume their ultimate identities. Beginning with her training with Christiane Nüsslein-Volhard in Germany, Ruth became fascinated by the most posterior region of the early Drosophila embryo, where the germ cells are the first cells to be specified. The cells form around membraneless structures called polar granules, a dense mixture of proteins and RNAs that are both necessary and sufficient for germ cell development. By targeting for study the maternal effect genes that are required for germ cell development, Ruth generated a beautifully coherent account of how the critical gene products of oskar, vasa, nanos, and tudor work in concert to generate polar granules. In the course of these studies, she discovered the importance of conserved RNA regulators that control the translation of mRNAs in the germline and are required to maintain germ cell identity in animals as distant from Drosophila as worms and mammals.
Once germ cells are specified in Drosophila, they must successfully migrate to the future gonad, where they will learn their ultimate fate: sperm or oocyte. By identifying genes that affect migration, Ruth’s lab painstakingly dissected the migratory pathway from beginning to end, showing that a G-protein coupled receptor in the germ cells is required to start the migration, and that lipid signals guide their journey to the gonad. When germ cells reach the gonad, Ruth showed that cell-cell communication between the germ cells and the resident somatic gonadal cells is necessary to finally signal that it is time to stop. Thus, like the little bunny who follows her nose, Ruth followed the development of germ cells wherever they led her, adopting new approaches and new technologies along the way, and making unexpected discoveries about Drosophila that were shown to be universal in the animal kingdom.
Like the germ cells she studies, Ruth Lehmann has migrated multiple times in her career. Following her training in Germany and the United Kingdom, Ruth began her independent career at the Whitehead Institute at MIT, where she became an Investigator of the Howard Hughes Medical Institute. In 1996, she moved to New York University as the Laura and Isaac Perlmutter Professor of Cell Biology and ultimately assumed the roles of the Director of the Skirball Institute of Biomolecular Medicine and Chair of the Department of Cell Biology. Just this year in the middle of a pandemic no less, she returned to MIT as the Director of the Whitehead Institute. Her list of honors is a long one, including the Edwin F. Conklin Award from the Society of Developmental Biology and the Keith Porter Award from the American Society of Cell Biology, professional societies for which she has served as president, and a lifetime achievement award from the German Society for Developmental Biology. Ruth is a member of the American Academy of Arts and Sciences and the National Academy of Sciences.
By choosing to honor these two brilliant and much admired scientists with the Amory Prize, the Academy is signaling its commitment to recognizing the highest standard of scholarship in life sciences. It is truly an honor to introduce them and to call them my friends.
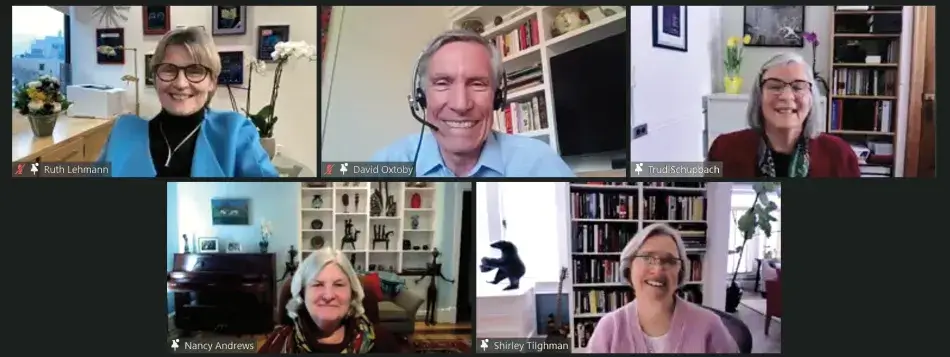
Gertrud Schüpbach is Emeritus Professor of Molecular Biology at Princeton University. She was elected a Fellow of the American Academy of Arts and Sciences in 1999.
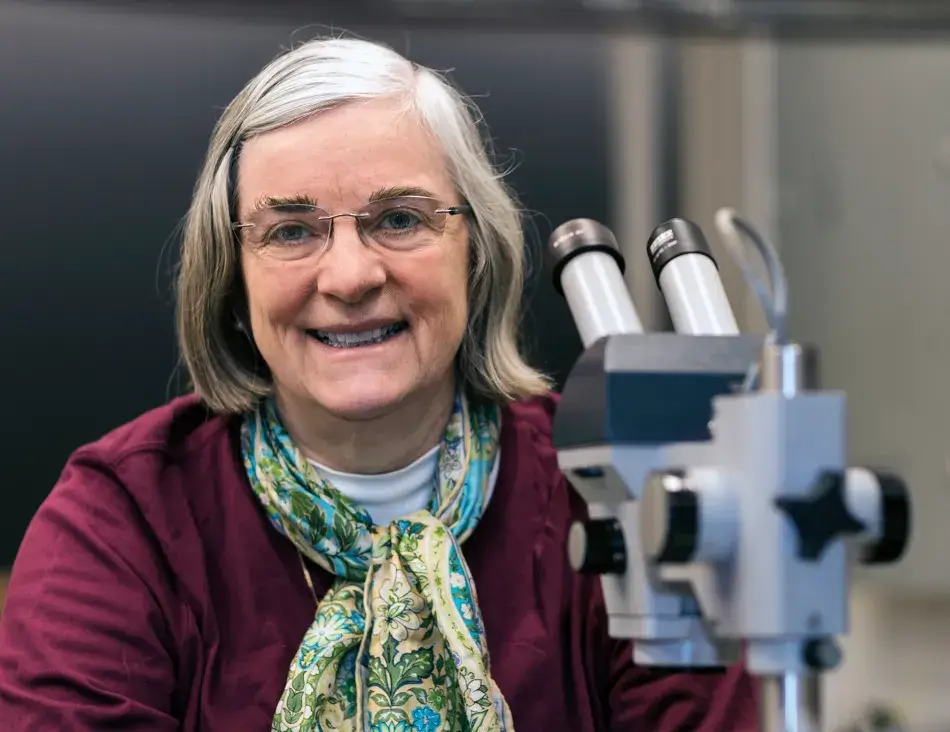
I would like to thank President Oxtoby, the prize committee, and the Academy for awarding us this prize. I am excited to be here and to share this honor with my longtime friend and colleague, Ruth Lehmann.
In my presentation, I want to give some insight into the research that has led to this moment. But before I get into the research, let me say something about my background. I was born and raised in Zurich, Switzerland. I attended the University of Zurich, where I was first introduced to questions of developmental biology. My Ph.D. thesis focused on the reproductive system and on sex determination in the fruitfly, Drosophila melanogaster. I was fascinated by the beautiful ovary of Drosophila, in which, as Shirley pointed out, eggs develop from stem cells in successive stages. And they do that in close proximity to somatic support cells.
Together with my partner, now husband, Eric Wieschaus, we moved to Princeton in the early 1980s, where we conducted a large-scale screen for mutations that would affect the egg or the embryo. Several overarching questions have guided my research, such as: How does an egg develop during oogenesis that then can support the development and patterning of an embryo? What factors are put into the egg by the mother during oogenesis that allow an embryo and eventually an adult to develop with a head at one end and a tail at the opposite end, and a dorsal and a ventral side? How much of the embryonic pattern is already programmed in the egg, and how does this happen?
Eggs are built during oogenesis, and they are surrounded by somatic support cells. This is true for all ovaries of higher organisms. In Figure 2, we see an egg chamber of Drosophila, and the follicle cells are surrounding the growing oocyte. These follicle cells will produce yolk and later will produce the eggshell. Oocytes are also connected to another type of helper cells, the nurse cells, which are synthetically very active and transport proteins and RNA into the oocyte. We can therefore predict at that early stage in the development of the egg chamber the anterior end of the egg and embryo by the position of these nerve cells, which connect to the anterior end of the oocyte. The future dorsal side of the egg and embryo is also already predictable because the oocyte nucleus is very asymmetrically positioned within the oocyte, and whichever side it is closest to will become the future dorsal side of the egg and embryo. This pattern is reflected in the eggshell. The two dorsal appendages that protrude from the egg indicate the region where the embryo will form its head because they indicate a dorsal anterior position within the egg.
Figure 1
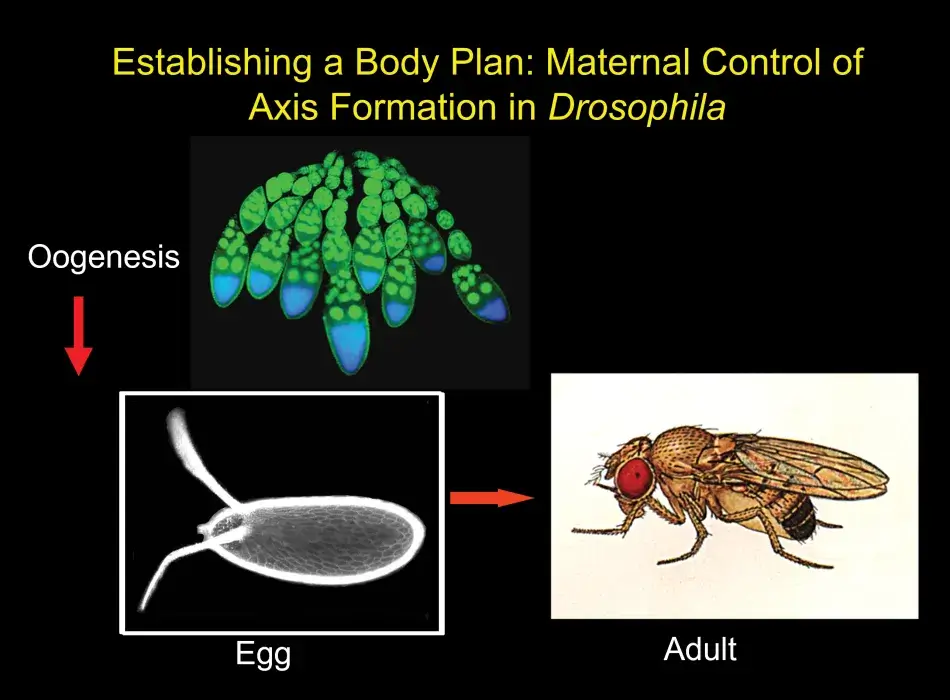
Figure 2
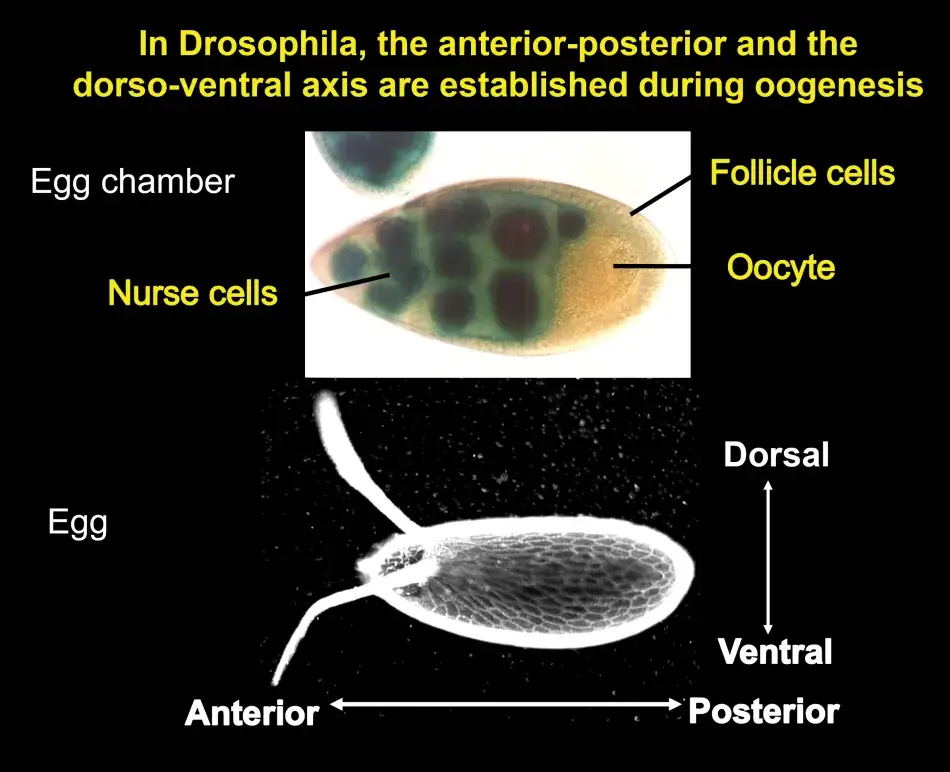
In order to find what factors were involved in generating these patterns, I conducted a screen for mutants that would show pattern alterations.
Figure 3 shows some examples. At the top is the wild type egg with two dorsal appendages. Below that is a ventralized egg and its dorsal appendages are lost. Follicle cells have formed ventral structures. At the bottom is a dorsalized egg, in which the dorsal structures are expanded at the cost of ventral structures. We found, and very excitedly so, that the embryos that develop inside such eggs are ventralized or dorsalized. This told us that we had made mutations in genes that are required for steps in pattern formation that have consequences both for the follicle cells as well as for the oocyte itself. This strongly suggests that there should be some sort of coordination between the oocyte and the follicle cells with respect to these patterns. Very talented postdoctoral fellows and graduate students who had joined my lab were able molecularly to clone the genes that had been mutated in these cases. And we found, to our excitement, that we had isolated mutations in a receptor and signaling pair. We also found very quickly that these genes had mammalian, and therefore human, homologs because the gene that we had called torpedo corresponded to the Drosophila EGF receptor, and the gene we had called gurken encoded a TGF-alpha like factor. These proteins are known from research in human cancer; the EGF receptor and its family members are involved in several different types of human cancers. Just as in humans, the Drosophila EGF receptor has several ligands that can bind to and activate the receptor. The one specific for oogenesis, Gurken, resembles the Transforming Growth Factor-alpha.
The molecular identification of these genes allowed us to ask where in the egg chamber these proteins are found. As we see in Figure 4, the EGF receptor outlined in white is present in all the follicle cells, presumably initially in an inactive form. In contrast, the ligand Gurken, which will activate the receptor on the adjacent follicle cells, is only found in this very restricted space above the oocyte nucleus. This allowed us to understand an aspect of pattern formation. Gurken will bind to and activate the EGF receptor in the adjacent follicle cells, and this induces them to assume a dorsal cell fate, in which they will express a certain set of genes. In contrast, the follicle cells on the ventral side see little if any Gurken and therefore the EGF receptor remains inactive and the cells enter a ventral cell fate, where they express a different genetic program.
Figure 3
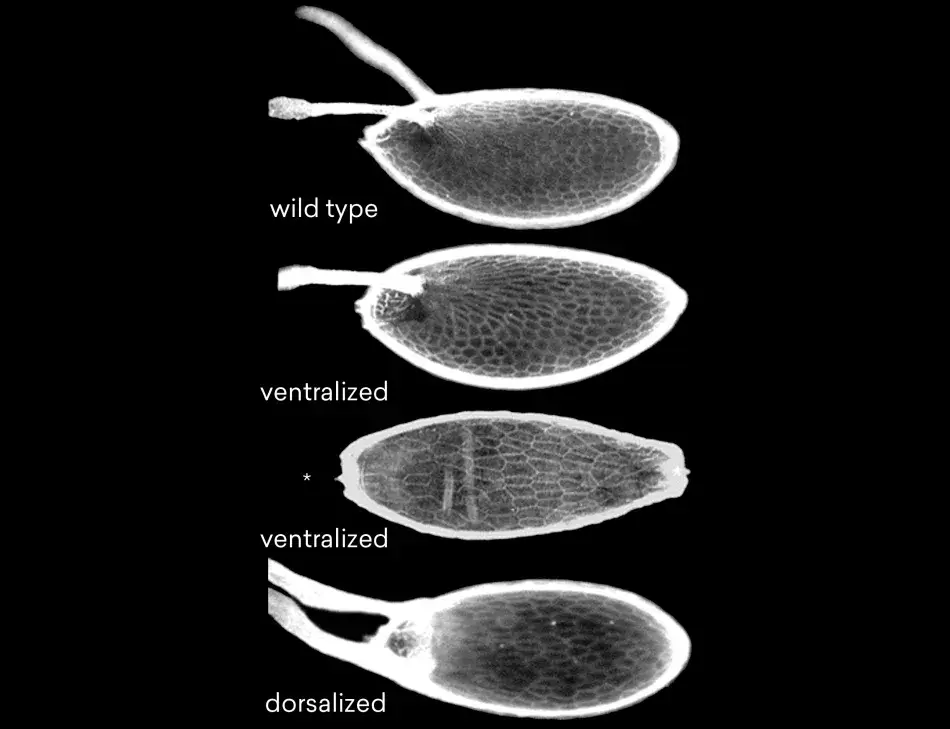
Figure 4
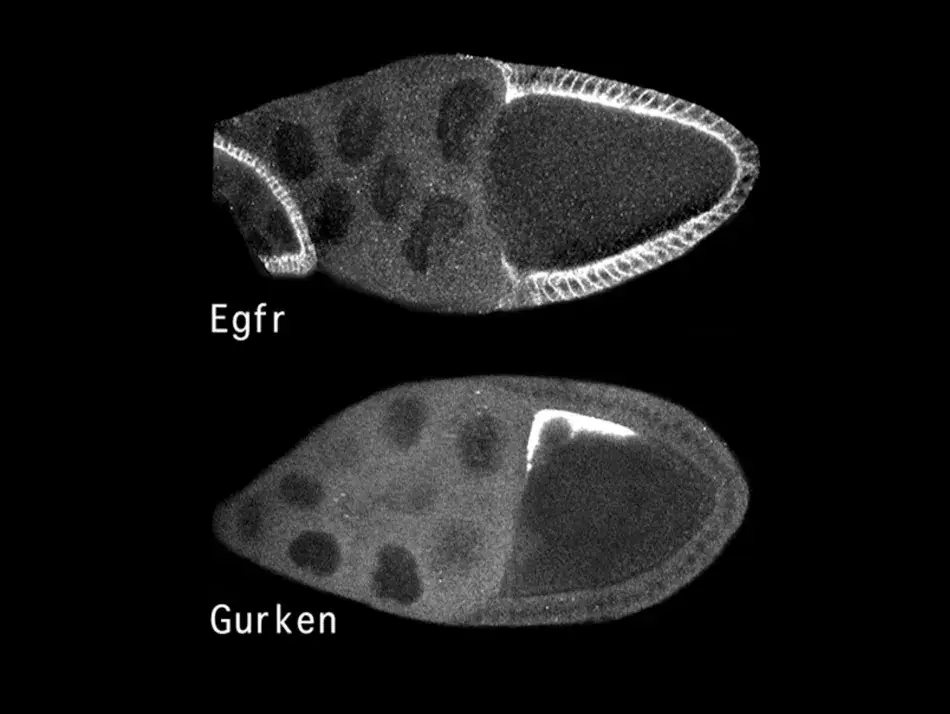
In science one result always raises many more questions. Why is the Gurken protein only found in this restricted area of the oocyte? We saw very soon that the process involved localizing the RNA that encodes gurken to this place. The RNA is transcribed in the nurse cells that are transported into the oocyte, and then anchored in the region around the oocyte nucleus.
The importance of the RNA localization became clear when we looked at mutants in which the gurken RNA wasn’t properly localized. In the mutant, the RNA is found all around the circumference of the oocyte (see Figure 5). This gives rise to mislocalization of Gurken protein and activation of the EGF receptor in all of the follicle cells. This results in an egg, in which all the follicle cells were activated to be dorsal, and therefore a whole ring of follicle cells produced this mass of dorsal appendage material.
Over time, we were able to define a pathway, where we found that there are several genes involved in the localization of the gurken RNA, some in the translation of the RNA into protein at just the right level at the right time. After the EGF receptor is activated in the adjacent follicle cells, the dorsal follicle cell program is initiated. In addition, this activity of the EGF receptor also represses a secondary signal that comes back from the follicle cells to the egg at a later stage, and which becomes important for the establishment of the embryonic pattern.
We see this illustrated in Figure 6, where we are looking at a cross section through an oocyte. The oocyte nucleus is at the dorsal side, and while not shown here, we know that the Gurken protein is activating the EGF receptor in the overlying follicle cells and eliciting these dorsal follicle cell fates in a gradient around the dorsal side. This activity represses the expression of this key factor, Pipe, which will later initiate a new signal that will induce ventral cell fates in the embryo that will be developing inside the egg.
Figure 5
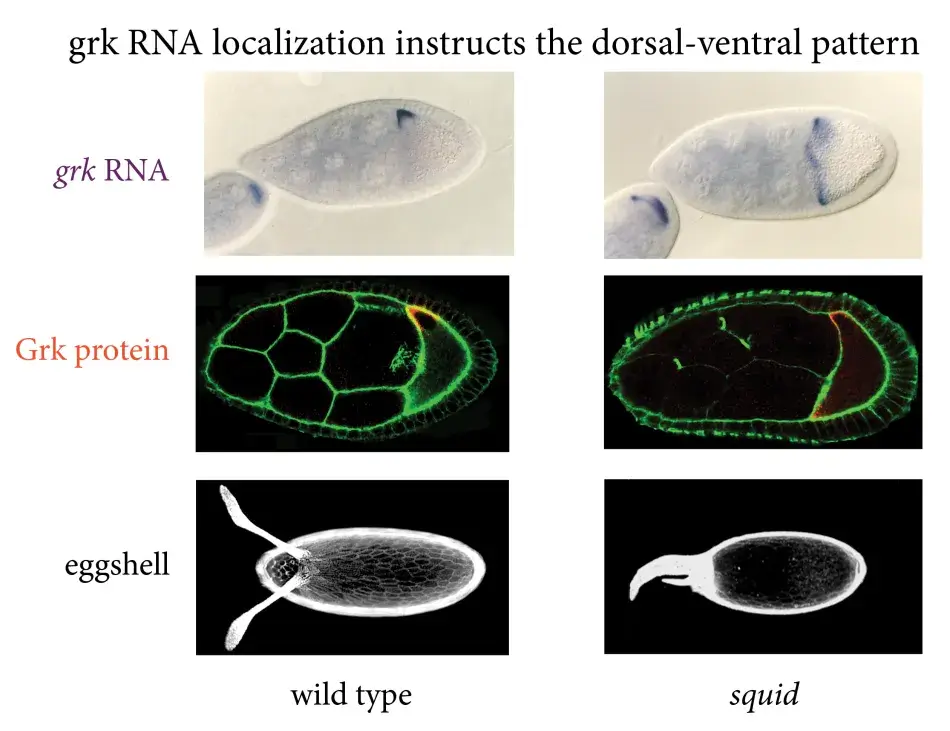
Figure 6
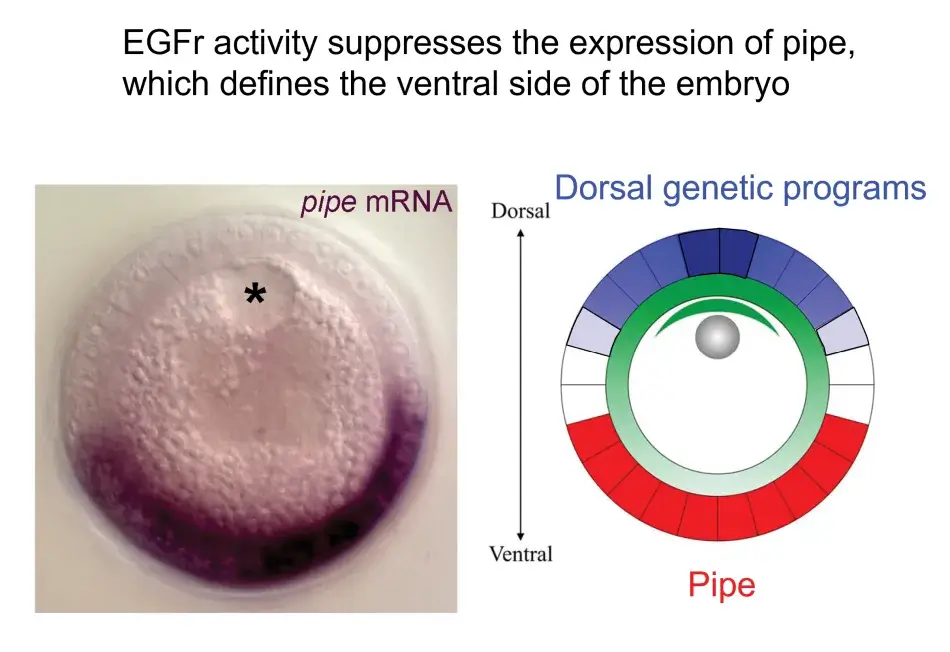
A little later, we discovered that the Gurken – EGF receptor signal was already operating at an earlier stage in oogenesis. In Figure 7, we have a young egg chamber. The oocyte is still very small, but Gurken protein is already visible within the oocyte (shown in green). Gurken activates the EGF receptor in the follicle cells and induces those follicle cells at the posterior to activate a specific genetic program that is different from the later dorsal or ventral program. These posterior follicle cells then signal back to the oocyte, and this results in the repolarization of the cytoskeleton inside the oocyte, which will become important for the anterior-posterior pattern of the embryo. The signal also causes the movement of the oocyte nucleus from an initially symmetric position to an asymmetric position. The gurken RNA and protein follow this movement and activate the EGF receptor in the cells on the lateral side and induce a dorsal cell fate.
This raises another question: how can the same ligand and receptor pair result in two different cell fates? This touches on a larger question in developmental biology. We know that there is only a limited number of signaling pathways that are active during development, and yet organisms have to establish hundreds of different cell types. How is this possible with this restricted number of signaling pathways?
To help us understand this posterior signaling process, we conducted more screens. And without going into the details here, we found that the posterior cell fate was affected not only by mutations in the EGF receptor pathway, but also by mutations in the Notch pathway (see Figure 8). Notch is another receptor that acts in many developmental programs. Putting a lot of data together, we found that at the early stage, there are, in fact, three different signaling pathways that are active in these posterior follicle cells. At the later stage, it is only the EGF receptor that sends a signal to the follicle cells and this single input then dictates dorsal follicle cell fate. Over time this analysis has shown us a beautiful picture of how in development a relatively simple organ, such as the egg chamber or the ovary, develops.
Figure 7
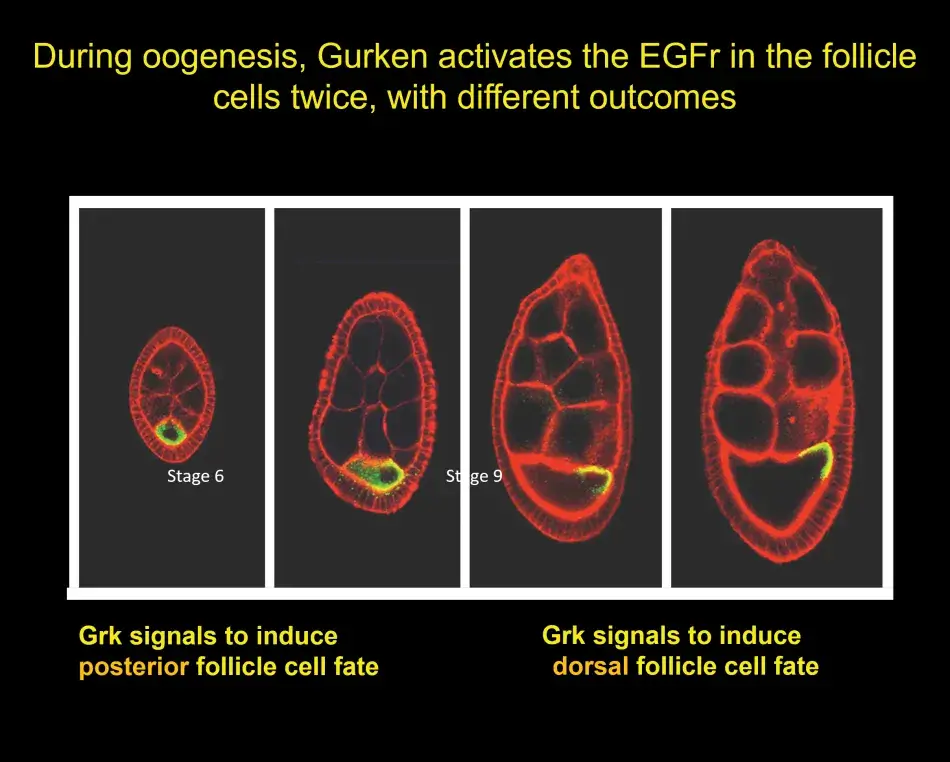
Figure 8
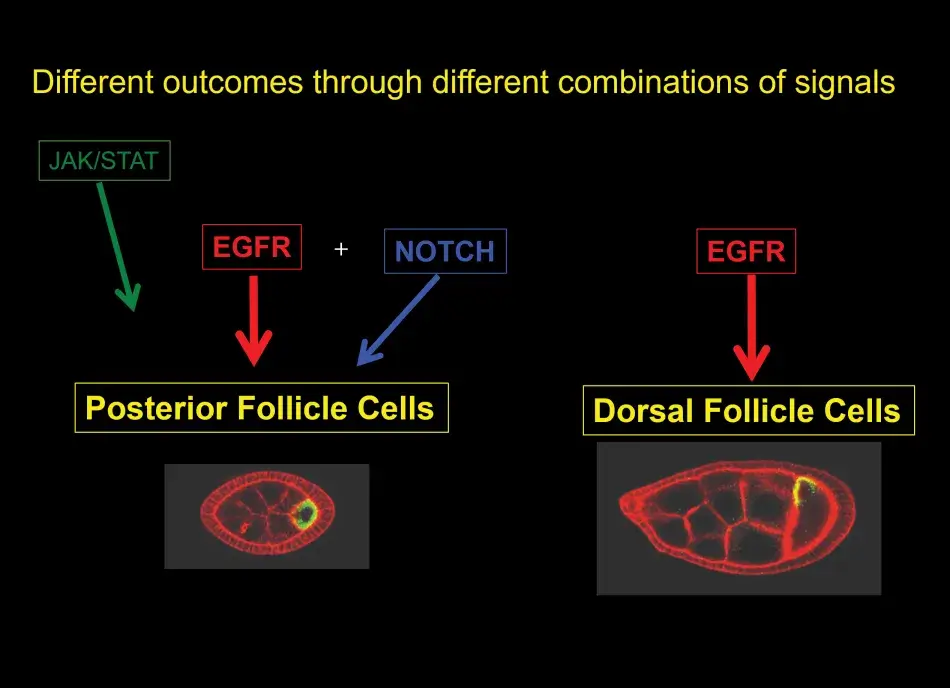
So what general lessons about signaling in development can we take away from the analysis with respect to the wide variety of cell types that make up an organism? First, we have learned that different cell fates can be achieved by combinations of different signaling pathways that are active at any one time. Second, we have seen that different cell fates can result from different levels of activation, for example, gradients of signaling molecules like Gurken determine a variety of different dorsal follicle cell fates. And third, I have not shown you this but we also learned that different cell types can be induced by modulating the duration of the signaling, and this is often regulated by feedback mechanisms.
In conclusion, I would like to thank all the people – postdoctoral fellows and students – who have worked in my lab and have contributed to this analysis over the years. I know that many of them have joined us today. I want to thank all of you again and say that it has been my great privilege and pleasure to work with all of you over the years. I also want to thank my longtime friends and collaborators, Eric Wieschaus and Stas Shvartsman. And finally, I thank the funding agencies that have supported my research over all these years.
Ruth Lehmann is Professor of Biology at the Massachusetts Institute of Technology and Director of the Whitehead Institute. She was elected a Fellow of the American Academy of Arts and Sciences in 1998.

I want to thank David Oxtoby and Shirley Tilghman for a spectacular review of our research. And I am grateful to Nancy Andrews for an overly generous recognition of our work. As we all know, you do not win these prizes from nowhere. There are always colleagues who have supported you along the way. I want to give a big shout-out and thank you to Hugo Bellen and Mitzi Kuroda. This was very unexpected, and I am incredibly honored.
I would like to share an overview of the germline. Let’s start with immortality. Obviously, we are mortal, but we have offspring and that offspring gives rise to new offspring and so on, and that is what I am referring to as immortality. The offspring is not identical to us. Rather, they are a combination of the paternal and maternal genomes. Germ cells are the cells that make sperm and egg, and thereby allow this immortality. Germ cells are destined to give rise to the next generation. Their naivety and their ability to rejuvenate within the organism are absolutely essential for the continuity of our species, but they themselves are dispensable for the development and function of the body.
Germ cells do all kinds of things. First, they have to be specified and set aside from all other cells in the body. Then they have to migrate to the somatic gonad, where they have to be protected not to develop into any other cell types or cell fates. And, finally, they have to be selected to be successful gametes, which means sperm or egg. I will touch on all these points because our work, to some extent more and to some extent less, has focused on the various phases of germ cell development.
We are studying – and this should not surprise you – germ cells in flies. Flies are a powerful model organism for genetic studies. My story starts with a genetic screen that you heard about from Trudi Schüpbach. Genetic screens were carried out for maternal effect mutations that affected the development, polarity, and segmentation of the embryo. The screen I was part of was carried out in Tübingen, Germany, in Christiane Nüsslein-Volhard’s lab. I was a starting graduate student in the lab with Hans Georg Frohnhöfer, another graduate student, and two postdocs, Kathryn Anderson and Gerd Jürgens. At the same time, Trudi Schüpbach and Eric Wieschaus were carrying out similar screens. There was a lot of back and forth because Eric and Trudi focused on mutations on the second chromosome and we were looking at the third chromosome.
One of the important findings from this screen was that the mother provided information through the egg to the embryo about its segmentation pattern: such as where to develop the head and tail and that the patterning information for the abdomen was linked to the origin of germ cells. This is illustrated in Figure 1, where we see an image of an embryo that was laid by a mother mutant for the oskar gene. The oskar gene defines the posterior group pathway. In oskar mutants as well as other genes of the same pathway, when the mother is mutant, the embryo will not have normal abdominal patterning, and it is also lacking the primordial (or early) germ cells at the posterior pole. After an analysis of the genetic pathway of the posterior group genes in Janni Nüsslein-Volhard’s lab, I started my own lab at the Whitehead Institute, where we identified the molecular nature of several posterior group genes, including the oskar gene. Anne Ephrussi, a postdoc in the lab, conducted a critical experiment: She had found that oskar RNA is localized to the posterior pole, but when she swapped the localization to the anterior pole, oskar alone was able to initiate the posterior pathway, and this pathway leads to the organization of the germ plasm. We now know that oskar is the key component that nucleates germ plasm.
So what is germ plasm? In Drosophila, germ plasm is formed at the posterior pole of the egg during oogenesis (Figure 2). It is a site where RNAs are localized. And these RNAs are localized within granules, which are composed of proteins in which Oskar is the starting point. These granules are membraneless and contain RNA, which is structured within the granules. The RNAs, which are localized to these granules, are important for many aspects of germ cell development. They are important for germ cell specification, like the nanos RNA, for their migration, and for their ability to escape the lethal differentiation into soma. These RNAs are specifically translated when they are localized. And they are protected in germ cells while they are degraded in the rest of the embryo that goes on to make soma.
Figure 1
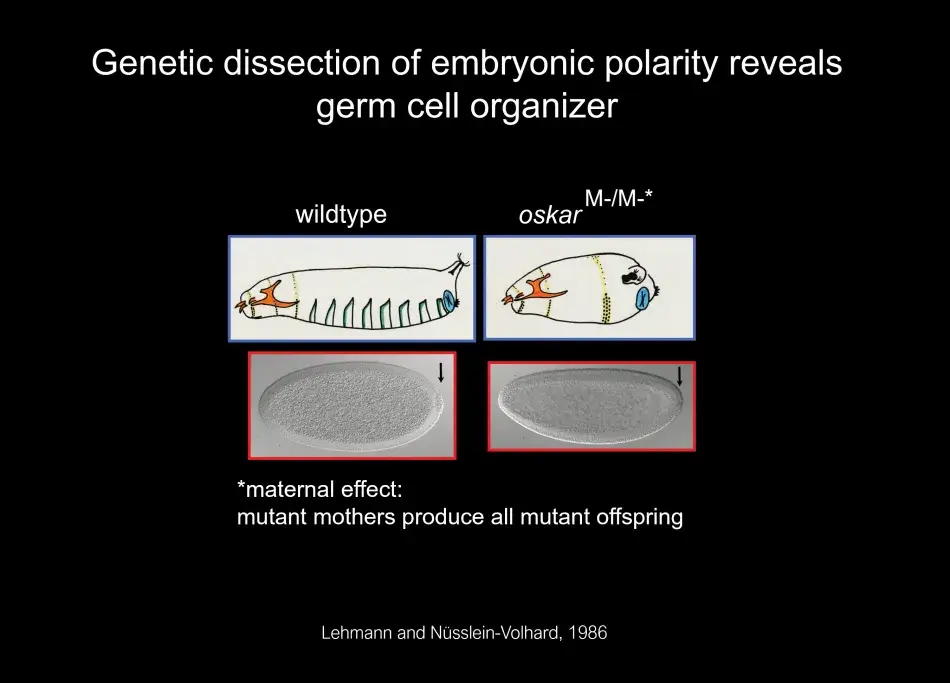
Figure 2

What we noticed was that with the germ plasm, RNAs segregate very specifically into germ cells as they form, and germ cells form earlier than any other cell in the body. Indeed, germ cells form by a completely different mechanism than all the other cells that will give rise to the soma (body) of the organism. Thus in the fly, the distinction between soma and germline occurs early and is very pronounced.
As part of this dichotomy, germ cells form at the fringe of the embryo, which is quite general for germ cell formation also in other species. At this time in development, the developing soma is actively transcribing and signaling, and you can see in Figure 3 a readout of this activity, with MAP kinase signaling at the termini promoting the development of head and tail structures. Germ cells, in contrast, remain naive by employing different molecular mechanisms to silence all somatic transcription and any transcription at all, and to degrade the particular signaling molecules, which could induce somatic differentiation. Thus, the first step in germ cell differentiation is to prevent being soma. Once germ cells have formed, they are not going to be able to make sperm and egg on their own. They first have to undergo a migration. They start directed migration as individual cells. On their way, they encounter various interactions with other cells, including repellents, and eventually they stop at the side of the somatic gonad where their differentiation into egg or sperm begins (Figure 4).
Figure 3
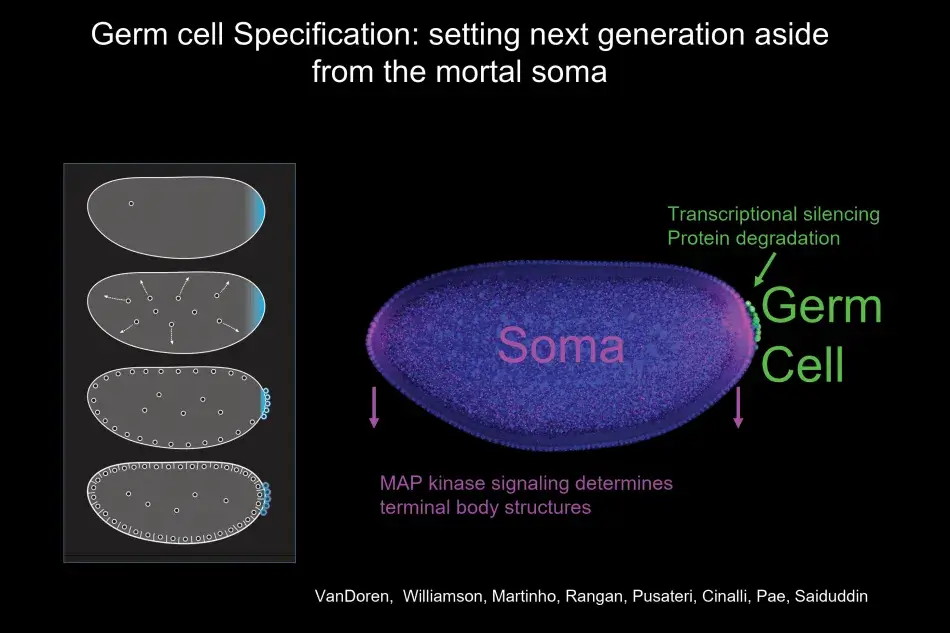
Figure 4
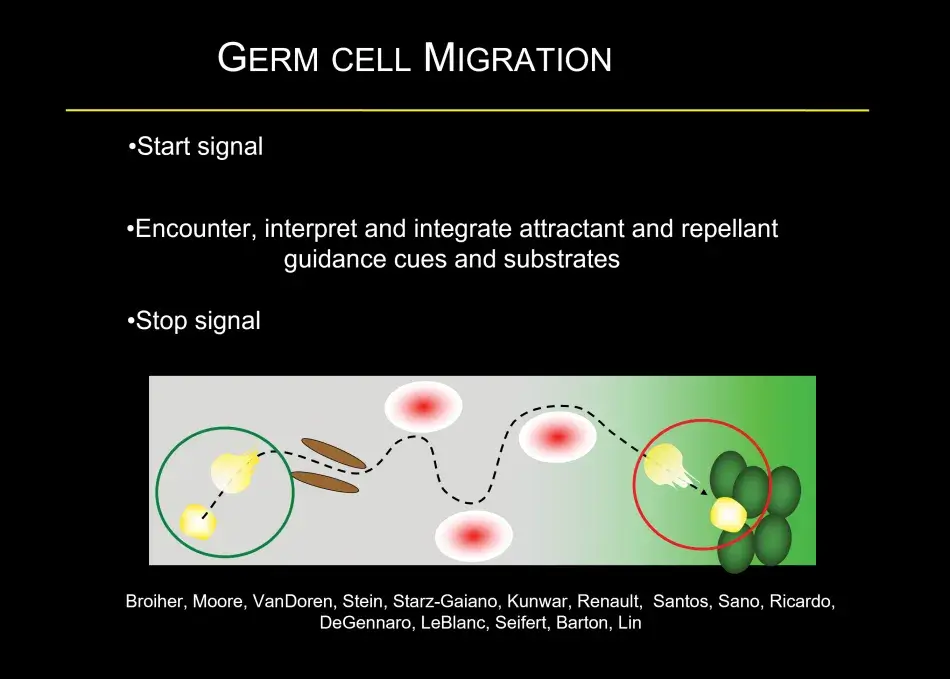
To give you a little bit of the flavor of this migration, this is quite common in many developmental models – germ cells form in a clutch – but most organisms have two gonads, so germ cells have to split into two on their migration and that is both mediated by repellent and attractive cues. Then germ cells have to find their way to the gonad, and that is associated, as we found recently, with a local hormonal signal from the gonad. Once the germ cells have made it to the gonad, they interact very closely with somatic cells. At this stage, germ cell sex is being determined, which will decide whether they are going to develop as an oocyte or a sperm, and interactions between the germ cells and the soma control how many germ cells there are. For example, if there are too few germ cells in the early embryo, they will catch up in number during the larval stages. During the larval stages, the ovary morphs from a ball of germ cells mixed with somatic cells into a highly sophisticated organ, the full-fledged ovary. Within the ovary, there are specific stem cell niches where stem cells are set aside, and in the adult female these stem cells will continue to generate egg chambers, which Trudi beautifully described (Figure 5).
Within the stem cell niche, there are two types of stem cells. There are germline stem cells, which will give rise to the egg. But there are also somatic stem cells, which give rise to all those follicle cells that are needed for the oocyte to grow but are also necessary for the prepattern of the oocyte to prepare for embryogenesis.
Both Trudi and I study the development of the egg, and as you already heard there is so much more to the egg than just the genetic information contained in the nucleus. For example, mitochondria are only transmitted through the egg and thus through the female germline. Mitochondria have their own genome. And that genome is very vulnerable as the mutation rate is high and it is not able to undergo recombination. So, variability between mitochondrial DNA molecules creates a heterogeneity that can cause mitochondrial diseases.
Almost one hundred years ago, in the 1930s, Muller proposed that without recombination there had to be some other mechanism of selection in organisms without sexual recombination, otherwise accumulation of mutations would lead to the death of the species. This concept is very relevant for mitochondria, because without selection mitochondria would accumulate mutations, leading to nonfunctional mitochondria unable to support the survival of the organism. What we found was that mitochondria are enriched in the region of the egg where the germ cells will form. These germ cells are endowed with a larger pool of mitochondria. The segregation of some mitochondria from the vast number of mitochondria in the oocyte to the germ cells is referred to as a bottleneck. The mitochondria that are sequestered in the germ cells will be the mitochondria of the next generation. We also identified when and how mitochondria are selected. We were able to point to the developing germline cysts, which are derived from the germline stem cells, as the stage where selection occurs. We analyzed the stage and mechanisms of selection by developing an RNA in situ hybridization protocol, which allowed us to distinguish between functional and nonfunctional mitochondrial genomes (Figure 6).
Figure 5

Figure 6
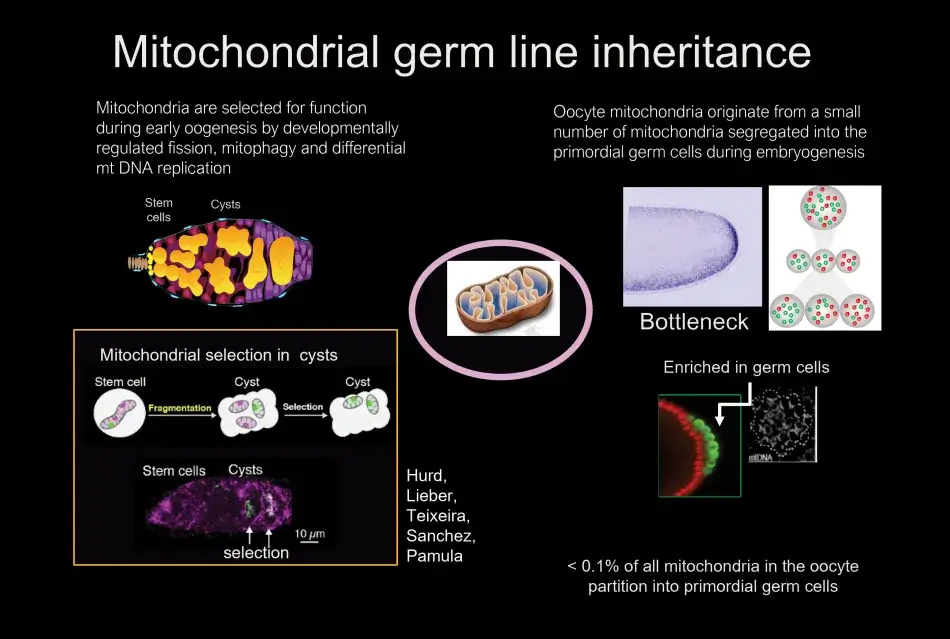
I will close by returning to an earlier question: what are the hallmarks of germ cells? Germ cells lack a master transcriptional regulatory program that determines their fate; instead RNA regulation is a fundamental and conserved principle underlying germ cell fate. Let me share two conclusions about the fundamental mechanisms that need to exist in germ cells to fulfill their unique function. One, there has to be genomic resilience so the genome can be passed from one generation to the next. As Trudi showed so beautifully, the successful completion of DNA recombination is read by the egg in order to determine whether it can form its normal pattern. So, if the recombination machinery is not working correctly at meiosis, patterning defects occur similar to those in gurken mutants. Part of the genomic resilience also requires that transposable elements, which can be active in the germline and may accelerate evolution, have to be controlled and if they cannot, this can be harmful for reproduction.
The second fundamental aspect of germ cell biology is that the egg is more than a nucleus; it provides the cytoplasm, including essential organelles such as mitochondria, for the next generation. In addition to mitochondria, there are symbionts like the bacterium Wolbachia in insects, which are carried through the female germline and can play important roles in reproduction but also as a defense against viruses. In summary, a combination of mechanisms that control genomic resilience and cytoplasmic maternal inheritance assure the continuity of the species.
The “we” that I have been referring to in my remarks are the many people who have worked in my lab. Over the years, they have contributed so much; they are incredibly smart, energetic, and resourceful. We had a reunion a few years ago, and that was very special for me. At one of our last gatherings, we were confined to sitting six feet apart in Washington Square Park. We hope to be together again in person soon.
I recently moved from the Skirball Institute at NYU Langone to the Whitehead Institute at MIT. I want to thank both institutions for supporting me so generously. I also want to thank my former and present funders. Finally, none of this would be possible without family, some of whom are watching from Germany, and my partner for many years, Steve Burden, and our dog Luke. And with that, I thank you again for this award. And my congratulations to Trudi.
© 2021 by Shirley Tilghman, Gertrud M. Schüpbach, and Ruth Lehmann, respectively
To view or listen to the presentation, visit www.amacad.org/events/award-lehmann-schupbach.