Imperatives for International Large-Scale Science
The benefits of international scientific collaboration for the United States and the world are substantial and eclipse the challenges they can present. This conclusion extends to engagement in large-scale science, when such engagement does not conflict with national security or other national goals. The CISP Large-Scale Science working group identified three principal reasons for the United States to engage in international large-scale science: 1) to enable inspiring discoveries that are otherwise impossible; 2) to improve lives; and 3) to promote international understanding. The following sections address each of these imperatives in turn.
Large-Scale Science Enables Inspiring Discoveries that Are Otherwise Impossible
On April 10, 2019, scientists working around the world made history when they captured the first image of a black hole and its shadow. Their achievement seized headlines and inspired wonder in the minds of people seeking to understand the universe around them.
The image was obtained following decades of worldwide investment in astronomy facilities and the use of eight radio telescopes in Chile, Spain, Mexico, Arizona, Hawaii, and Antarctica for a week-long observation in April 2017.6 Without this concerted, international effort, this scientific achievement would have been impossible.
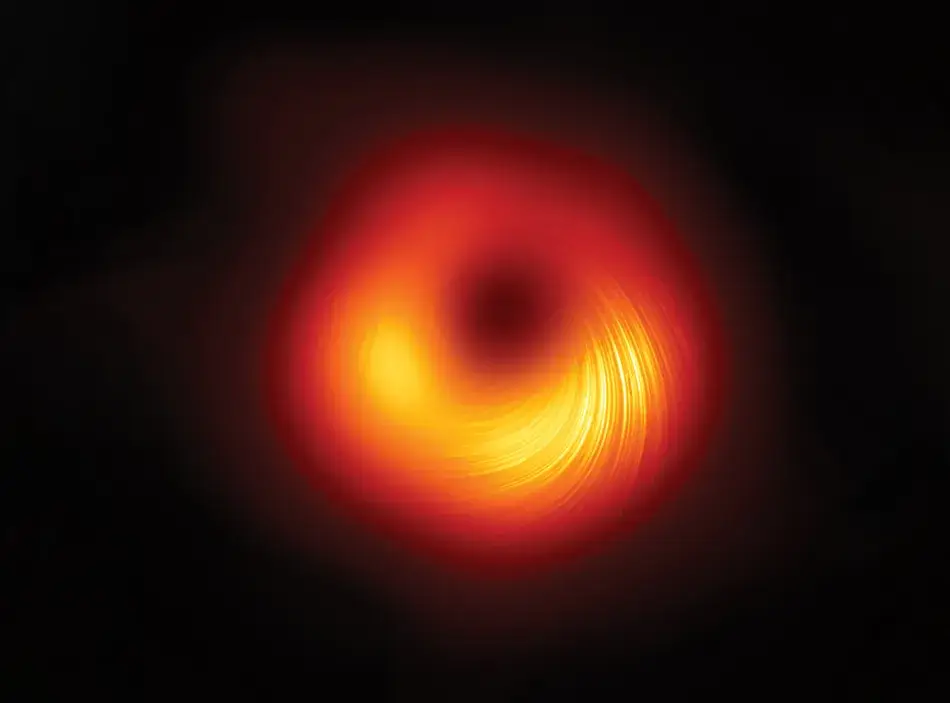
The Event Horizon Telescope utilized radio telescopes positioned across continents to capture the first image of a black hole, a supermassive black hole at the center of Galaxy M87, shown here with a view in polarized light. Image courtesy of the Event Horizon Telescope Collaboration.
On August 17, 2017, the Laser Interferometer Gravitational-Wave Observatory (LIGO) Scientific Collaboration (LSC) detected gravitational radiation from a coalescing pair of neutron stars and, combined with observations by its European counterpart, Virgo, as well as detection of a short gamma-ray burst by NASA’s Fermi Gamma-ray Space Telescope, localized this source in a distant galaxy.7 The two NSF-funded LIGO detectors are based in the United States, but an international collaboration of more than one thousand scientists from eighteen countries made key contributions to the U.S. LIGO interferometers. U.S. LIGO scientists collaborate with scientists operating other detectors located around the world and funded by other nations.8 The international detector locations allow scientists to take advantage of detection delays across facilities to pinpoint gravitational wave source locations, as well as reduce noise from local sources close to individual detectors.9 Observations by optical observatories around the world of the electromagnetic radiation produced by the merger event directly showed that these mergers are important producers of heavy elements, including gold, thus addressing the long-standing scientific question of the origin of these elements.
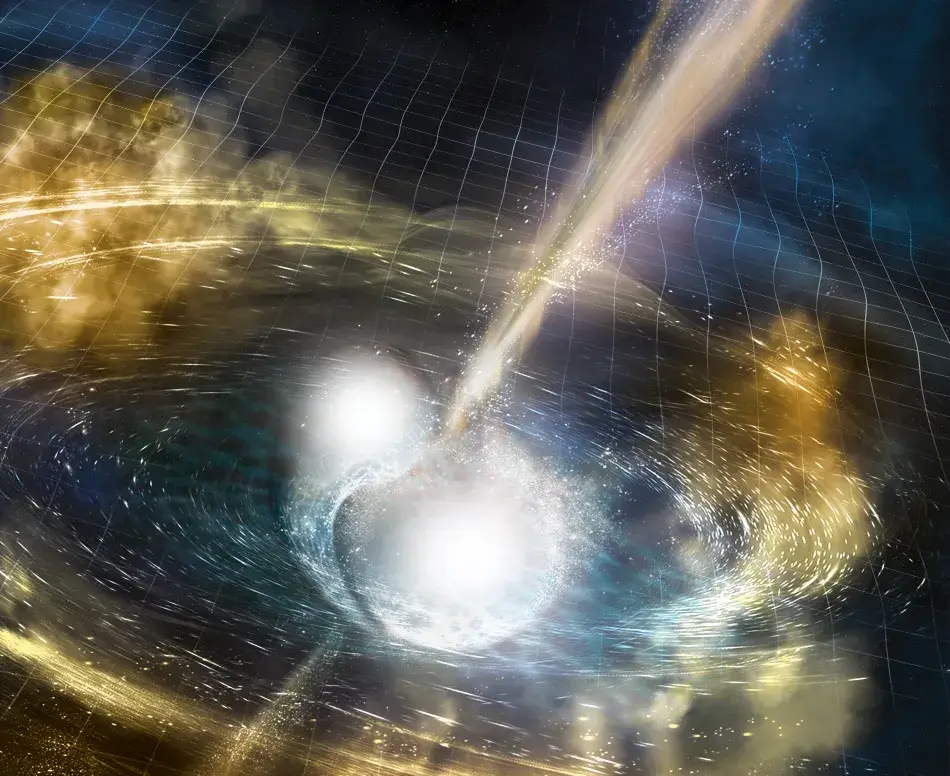
Artist’s illustration of two merging neutron stars. The rippling space-time grid represents gravitational waves that travel out from the collision, while the narrow beams show the bursts of gamma rays that are shot out just seconds after the gravitational waves. Swirling clouds of material ejected from the merging stars are also depicted. The clouds glow with visible and other wavelengths of light. Illustration by Aurore Simonnet. Image courtesy of National Science Foundation, Laser Interferometer Gravitational-Wave Observatory, and Sonoma State University.
Achievements such as these, which represent just a few of the major milestones reached across scientific disciplines by international megascience, capture the imaginations of people around the globe. They are crowning examples of what humanity can accomplish through imagination, cooperation, and long-term investment in large-scale international endeavors.
Large-Scale Science Improves Lives
Beyond satisfying a human desire to unlock the world’s mysteries, large-scale scientific endeavors often require pushing beyond the limits of existing technology and yield major advances that become important for society as a whole (see Using Synchrotron X-Rays to Accelerate Discovery of New Therapeutics).
Genomic Research
In 1990, the NIH and DOE submitted their initial five-year plan for what was, at that time, an enormous undertaking: sequencing the human genome. The two agencies had formalized their relationship in this endeavor in a memorandum of agreement and articulated the benefit of international collaboration to the United States:
The Human Genome Initiative is not limited to the United States. Many countries are interested in participating in the project and all are interested in the outcome. . . . There are many opportunities where international collaboration could enhance progress on the Human Genome Initiative. Currently, the United States is in a leadership position with respect to scientific accomplishment and organization of the genome program. However, as other nations organize and initiate their programs, the United States will stand to gain by international collaboration as much as the other countries involved.10
In the years that followed, the Human Genome Project (HGP) was undertaken by the International Human Genome Sequencing Consortium, which comprises sequencing centers in the United States, United Kingdom, Japan, France, Germany, and China.11 The effort was predicted to take fifteen years and cost $3 billion in 1991 ($5.7 billion today), but was accomplished two years early and under budget.12 All of the sequence data were made public and freely available without restriction on use; they represented 99 percent of the gene-containing sequence of human DNA.
The impact of this project has been monumental. The HGP revealed approximately 20,500 human genes and enabled the characterization of genomes from other organisms, such as mice and fruit flies, that are commonly used in biological research.13 It revolutionized the biomedical community, accelerating the discovery of genes associated with disease and, in turn, providing improved diagnoses and treatments. The HGP is also estimated to have brought significant economic benefits to the United States, although the magnitude of this benefit varies in analyses conducted thus far.14
The technology and the reference genome have become the cornerstone of many ongoing international collaborative efforts. One, the International Nucleotide Sequence Database Collaboration, links three databases from the United States, United Kingdom, and Japan that collect DNA and RNA sequences and synchronize them for use by researchers.15 Most recently, this collaboration supported global research on the SARS-CoV-2 virus by providing a streamlined, global bioinformatics system that worked to promote rapid, maximally impactful sharing of viral sequences.16 Another, the Human Cell Atlas, includes scientists and institutes in more than seventy countries. This collaboration is using single-cell DNA and RNA sequencing, among other techniques, to identify new cell types and create a reference map of all human cells, potentially identifying novel targets for disease treatment.17
Particle Accelerators
Particle accelerators and their associated detectors and computing facilities, such as those at the European Organization for Nuclear Research (CERN), provide a clear example of large-scale science focused on probing nature on subatomic scales, leading to advances in technology and expertise that contribute to applications in fields beyond high-energy physics and address global challenges in areas such as health and environment.18 These facilities produce beams of charged particles using electric fields to accelerate the particles to high speeds and energies and are essential for many particle and nuclear physics experiments, including those that led to the observation of a new particle identified as the Higgs boson.19 The advancements made in particle accelerators, detectors, and associated computing have transformed everyday life. First, they have improved critical technologies used across society, including the manufacturing of computer chips, the inspection of nuclear fuels, X-ray diagnostics, and radiation therapy for cancer patients.20 Second, they have provided the collaborating nations with economic growth through the development of industrial capabilities related to those nations’ contributions to the CERN experiment.
Synchrotron Radiation
Following directly from the development of particle accelerators, synchrotron light source facilities, producing X-rays that are ten million times more intense than those from an X-ray tube, are increasingly constructed by international partnerships. There are now over seventy synchrotron facilities around the world carrying out a range of experiments. The heart of a synchrotron light source is a ring of magnets into which a beam of high-energy electrons, produced by an accelerator, is injected and stored. The circulating electrons emit synchrotron radiation that is used to probe the structure of matter from subnanometer to millimeter scales. The extreme intensities and other characteristics of these facilities make possible transformational investigations in many fields, including materials science, physics, chemistry, structural biology, and medicine. Several Nobel Prizes have been awarded for research that depended on synchrotron light. An example of current research facilitated by synchrotron light sources is the study of the SARS-CoV-2 virus (see Using Synchrotron X-Rays to Accelerate Discovery of New Therapeutics). These facilities play an increasingly important role in the international science effort needed to address challenges such as pandemics.
Indirect Benefits of Large-Scale Science
Large-scale facilities commonly produce major technological advances seemingly unrelated to their primary scientific goals: for instance, the World Wide Web was developed at CERN in 1989 when Tim Berners-Lee worked to enable the sharing of data and scientific information among a globally dispersed group of collaborators.21 Pushing the frontier of knowledge itself has meant that the scientific advancements, such as those made in the development of LIGO, have accelerated or invented numerous technologies, including in the fields of quantum measurement science, materials science and optics, and cryogenics.22
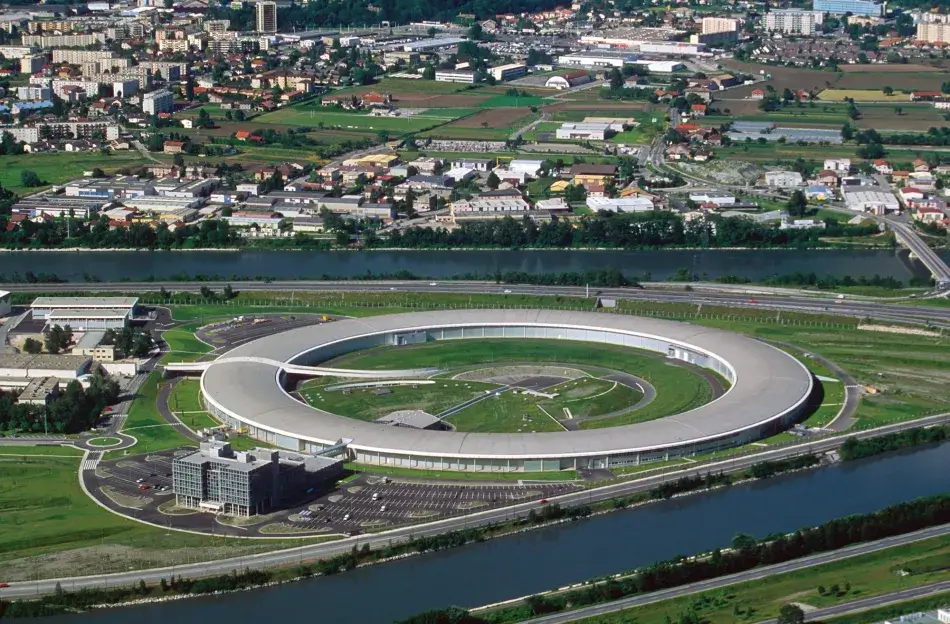
The European Synchrotron Radiation Facility (ESRF), located in Grenoble, France, is a partnership of twenty-two nations, of which thirteen are members and nine are scientific associates. The ESRF hosts more than six thousand researchers that use forty-two beamlines at the facility. © by gui00878/Getty Images.
As this report goes to print, the COVID-19 pandemic continues to damage the health, well-being, and economy of every nation. There are more than 159 million confirmed cases worldwide, accompanied by more than three million deaths. Both near- and longer-term strategies for controlling the pandemic and mitigating its impacts on human health depend in part on the discovery of new therapeutics to defeat it and vaccines to prevent its spread. Being able to look at the intimate workings of the virus in molecular detail is an essential element of the strategy to rapidly develop and deploy such new pharmaceuticals. Because of the very small size of the virus particles (~0.12 microns), optical light is not effective in imaging their even smaller molecular components, so scientists use other imaging techniques based on X-rays or electrons. X-rays in particular have proven over decades to provide invaluable structural information for guiding the development of new therapeutic approaches, from curing cancers to controlling viral infections such as HIV, SARS, and Ebola.
Such molecular imaging studies depend substantially upon access to brilliant sources of X-rays that are produced in synchrotron light sources. The scale and cost of these machines dictate that they are operated as national or international scientific user facilities. Most of the approximately seventy synchrotron facilities, located in over twenty countries, have active research programs enabling the study of viruses and their components.23 There are five large-scale synchrotron laboratories in the United States supported primarily by the Department of Energy and one by a consortium of private and public sources.24 International cooperation among synchrotron light sources is a well-established and important means of providing the most advanced capabilities for the international user community, which is estimated to be in excess of fifty thousand.
The synchrotron X-ray sources enable very rapid, precise studies of molecular details in complex biomolecules. Biologists have determined more than 120,000 3D protein structures from synchrotron X-ray source measurements.25 These high-resolution structural insights into viruses and other macromolecules help guide the identification of potential drugs and antibodies and, through understanding of how they work, guide their further development.26
Each synchrotron X-ray facility is an ecosystem in which techniques for scientific investigation are developed and maintained at the state of the art to serve a large community of academic, research laboratory, and industrial users. Such facilities are well positioned to respond to rapidly emerging threats and national needs, as exemplified in the current COVID-19 pandemic. As seen at many of the synchrotron laboratories worldwide, within weeks of widespread “shelter in place” orders to control the spread of the virus, priority was given to reopening and operating these facilities because of their essential role in COVID-19–related research. Safe laboratory operations procedures were developed and implemented quickly, and light source research has led to significant progress against the challenges of COVID-19.27
Gaining a molecular level understanding of every aspect of the SARS-CoV-2 virus, the virus responsible for the COVID-19 pandemic, is essential. Studying its life cycle, including how it infects cells, replicates, and multiplies, offers the potential for evolving existing proven therapeutic strategies developed for other viruses and developing new ones for stopping the infection of SARS-CoV-2 in humans. Experimental studies, coupled with advanced computational tools, are already accelerating discoveries of new therapeutics for defeating the SARS-CoV-2 virus and providing the strategies and key infrastructure to rapidly address future drug resistance challenges that will emerge.
Two recent studies, both enabled by X-ray synchrotron radiation crystallography, illustrate complementary approaches to rapidly developing effective therapies for COVID-19. One of the studies, by biologist Ian A. Wilson and colleagues at Scripps Research, has provided remarkable molecular insights into the process of how antibodies recognize the spike proteins on the SARS-CoV-2 virus surface, leading to the design of an experimental vaccine.28
The second study, by researchers at the University of Alberta, also facilitated by synchrotron X-rays, investigated a complementary strategy for defeating the SARS-CoV-2 viral infection process by blocking viral replication once it has entered the human cell.29 The researchers used X-ray crystallography to learn how a candidate drug (and variants of it) interacts with one of the protease enzymes involved in viral replication within the infected cell, much as a key fits into a lock and disables it, preventing the virus from making copies of itself.
While these examples illustrate the quick deployment of synchrotron X-ray techniques to enable rapid development of new therapeutics to help treat and prevent COVID-19, there are other areas of research in which synchrotron radiation is playing a role. These include using X-rays to understand how mask materials, such as those used in N95 respirators, function at the molecular level, how they can be effectively decontaminated, and how improved materials can be designed. An example of a national network focused on all of these approaches is the National Virtual Biotechnology Laboratory (NVBL), which is a consortium of DOE national laboratories coordinating a response to COVID-19.30 The NVBL coordinates COVID-19–related research that takes advantage of the DOE laboratories’ capabilities, including their synchrotron light sources.
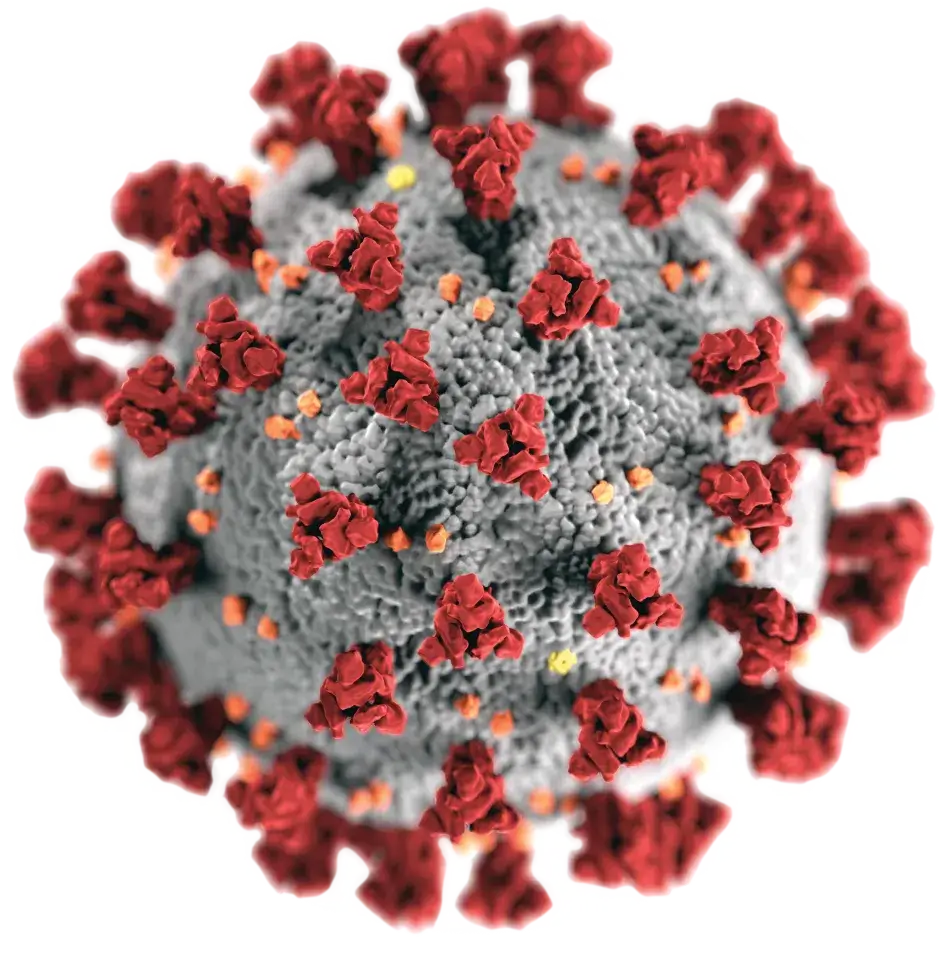
Illustration of the structure of the SARS-CoV-2 coronavirus. Colored in red are the spike proteins that are key in recognizing and infecting the human host cells. Image by Centers for Disease Control and Prevention.
Large-Scale Science Promotes International Understanding
International large-scale scientific facilities and networks provide opportunities to promote understanding between different nationalities and nations, including nations that have strained diplomatic relations. CERN is an important example.
In the post–World War II Cold War era, CERN was one of the few places in the world where Soviet, European, and U.S. scientists worked side-by-side. From its founding in 1954 by twelve European nations, part of CERN’s mission has been to “unite people from all over the world to push the frontiers of science and technology, for the benefit of all.” CERN’s charter states: “The Organization shall have no concern with work for military requirements and the results of its experimental and theoretical work shall be published or otherwise made generally available.”31 From its start, CERN adopted a policy of open access. Since 1954, the number of member states has grown to twenty-three nations, with an additional eight associate member states. Japan, Russia, and the United States formally have observer status at CERN, while an additional thirty-five nations, including China, Egypt, Iran, Jordan, and Saudi Arabia, have signed cooperation agreements with the organization. CERN is run by the CERN Council, comprising two representatives, one a scientist and one a government delegate, from each of the twenty-three member states. The financial viability and stability of CERN derive from its founding structure, which is based on intergovernmental treaties.
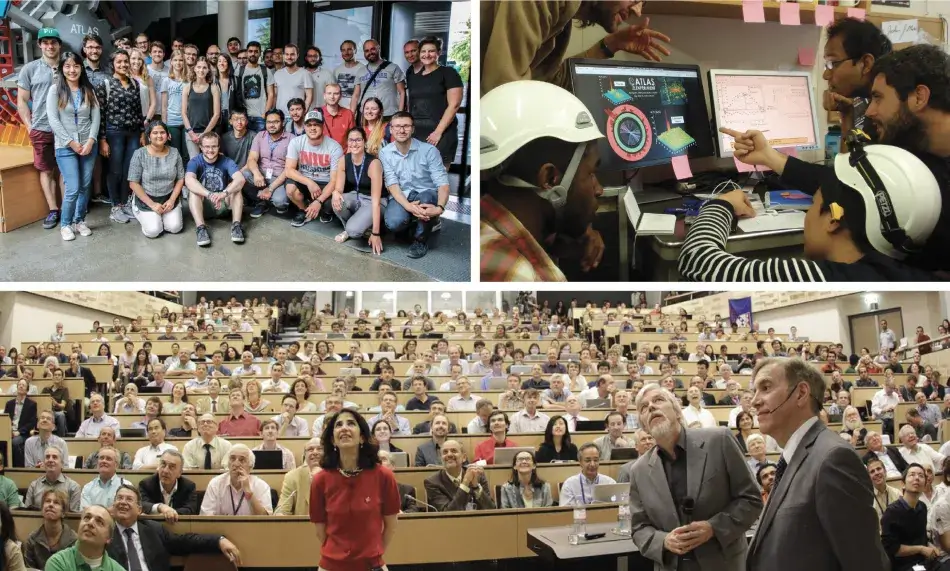
“People from all over the world come here, bringing with them different cultures and different ways of working. This diversity is part of our strength, and it’s something that we need to nurture constantly.” —Fabiola Gianotti, CERN Director-General.32 © by the European Organization for Nuclear Research (top row) and AP Photo/Denis Balibouse (bottom).
CERN’s on-site staff of 2,500 people from around the world are responsible for the design, construction, and operation of CERN’s research infrastructure. They also support the preparation and operation of the experiments as well as the data analysis infrastructure for a user community of more than 12,200 scientists of 110 nationalities from institutions in more than 70 countries.
U.S. participation in CERN experiments has been governed in recent years by the 2015 DOE-NSF-CERN International Cooperation Agreement and subsequent protocols.33 Since 1997, DOE and NSF support for the massive ATLAS and CMS detectors has been implemented via NSF and DOE funding to U.S. universities and national laboratories to develop, operate, and maintain specific components of these detectors. Further, the 2015 agreement paved the way for additional U.S. contributions toward an upgrade to the Large Hadron Collider (LHC) that will enable the instruments to function at much higher collision rates.34 This mode of participation allows U.S. researchers to be active members of the LHC experiments, even though the United States does not take on all the obligations of full CERN membership.
CERN became a model for the formation of other successful scientific partnerships among European nations, including the European Southern Observatory (ESO) in 1962, the European Molecular Biology Laboratory (EMBL) in 1974, and the European Synchrotron Radiation Facility (ESRF) in 1988. Most recently, the Synchrotron-light for Experimental Science and Applications in the Middle East (SESAME) was built in Jordan in 2002 under the auspices of UNESCO, following the CERN model, and lists its current members as Cyprus, Egypt, Iran, Israel, Jordan, Pakistan, Palestine, and Turkey.35
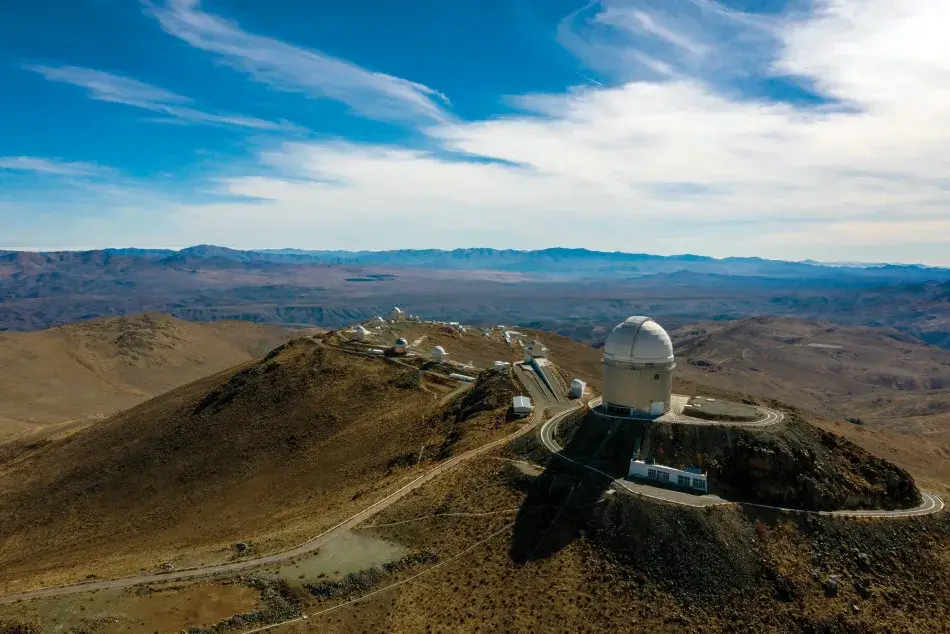
The European Southern Observatory’s La Silla facility in La Higuera, Coquimbo Region, Chile. Photo by Martin Bernetti/AFP via Getty Images.